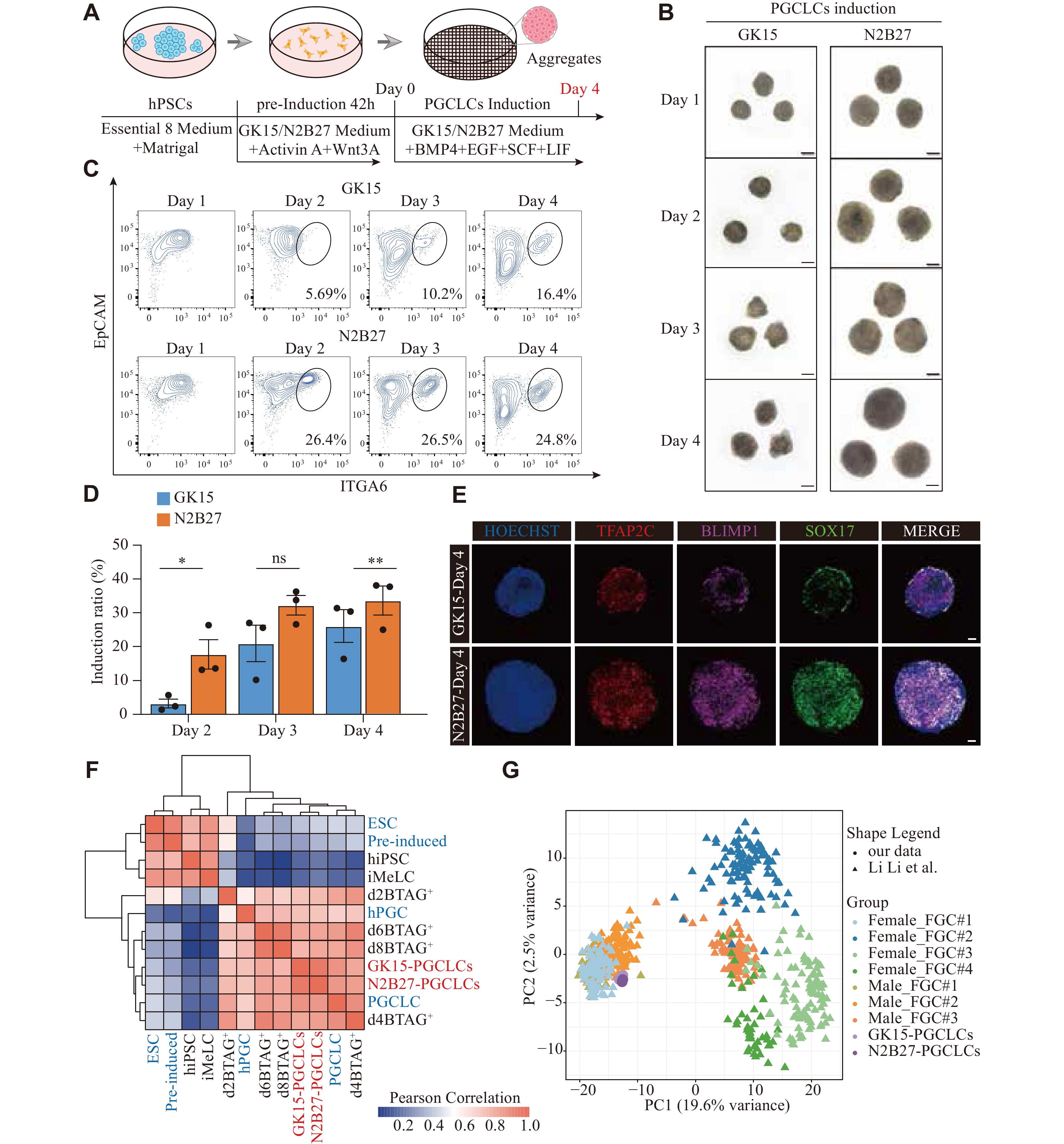
Citation: | Gege Yuan, Jiachen Wang, Shuangshuang Qiu, Yunfei Zhu, Qing Cheng, Laihua Li, Jiahao Sha, Xiaoyu Yang, Yan Yuan. Improving in vitro induction efficiency of human primordial germ cell-like cells using N2B27 or NAC-based medium[J]. The Journal of Biomedical Research. DOI: 10.7555/JBR.38.20240433 |
Unproofed Manuscript: The manuscript has been professionally copyedited and typeset to confirm the JBR’s formatting, but still needs proofreading by the corresponding author to ensure accuracy and correct any potential errors introduced during the editing process. It will be replaced by the online publication version.
Primordial germ cells (PGCs), the precursors of oocytes or spermatozoa, are highly pluripotent. In recent years, the in vitro induction of human primordial germ cell-like cells (hPGCLCs) has advanced significantly. However, the stability and efficacy of obtaining hPGCLCs in vitro still require further improvement. In the current study, we identified a novel induction system by using Dulbecco's Modified Eagle Medium/Nutrient Mixture F-12 (DMEM/F-12) as the basal medium supplemented with B27 and N2 (referred to as N2B27) in combination with four cytokines: bone morphogenetic protein 4 (BMP4), stem cell factor (SCF), epidermal growth factor (EGF), and leukemia inhibitory factor (LIF). The hPGCLCs induced under these conditions closely resemble PGCs from 4 to 5-week-old embryos at the transcriptome level. Compared with traditional GK15 (GMEM supplemented with 15% Knockout™ SR)-based induction conditions, the N2B27 system significantly increased the speed and efficacy of hPGCLC induction. RNA sequencing analysis revealed that this improvement resulted from an increased cell capacity to cope with hypoxic stress and avoid apoptosis. The N2B27 medium promoted an increase in mitochondrial activity, enabling cells to better cope with hypoxic stress while also reducing the production of reactive oxygen species. Moreover, by gradient concentration experiments, we demonstrated that addition of the common antioxidant N-acetyl-L-cysteine at an optimized concentration further enhanced the efficiency of PGCLC induction under GK15 conditions. Thus, our study established an optimized induction system that enhances the efficiency of hPGCLC differentiation by improving cellular resilience to hypoxic stress and apoptosis.
Primordial germ cells (PGCs) are precursor germ cells that emerge during early development and eventually give rise to mature sperm and oocytes[1-3]. PGCs first appear in the embryo around day 12 and subsequently migrate to the gonadal ridge, where they undergo further differentiation and proliferation, ultimately leading to the formation of mature germ cells[4]. Research on PGCs is extremely crucial for improving our understanding of germ cell development and the mechanisms underlying infertility[5,6].
Significant progress has been made in the study of mouse primordial germ cell-like cells (mPGCLCs), both in terms of system development and applications[7-10]. In recent years, the induction of human primordial germ cell-like cells (hPGCLCs) from pluripotent stem cells (PSCs) in vitro has also advanced considerably. Using factors, such as bone morphogenetic protein 4 (BMP4), stem cell factor (SCF), epidermal growth factor (EGF), and leukemia inhibitory factor (LIF), and methods, such as two-step induction and direct induction, investigators have obtained large quantities of seed cells for studying germ cell development[11-13]. However, various methods exhibit instability, possibly due to the uncontrolled plasticity of PSCs in response to cytokines and the inherent limitations often encountered in in vitro experiments.
PSCs, particularly those related to hypoxic stress, face significant challenges during the in vitro culture[14]. In contrast to the in vitro normoxic conditions (21% O2), the in vivo environment of PSCs is generally hypoxic, with oxygen levels ranging from 1% to 5%[15-17]. Culturing PSCs under normoxic conditions can induce oxidative stress, disrupting cellular metabolism and mitochondrial function[18]. Such an imbalance can lead to the accumulation of reactive oxygen species (ROS), which, if left unchecked, can cause oxidative damage. This increase in ROS impairs the proliferation, differentiation, and self-renewal of PSCs[19]. Therefore, it is essential to optimize the in vitro microenvironment to better mimic the physiological conditions of PSCs, to reduce oxidative stress and preserve their functional integrity. Especially, maintaining a balanced oxygen level and antioxidant presence in the culture system is crucial for ensuring the stability of PSC-related experiments.
In the current study, we developed a novel induction system in which the N2B27 medium enriched with antioxidant components, was used to induce hPGCLCs. By comparing the hPGCLC production under the N2B27 and original GK15 induction conditions, we found that antioxidant components effectively mitigated some oxidative stress challenges faced by PSCs in vitro, thereby increasing the efficacy of hPGCLC induction. Additionally, the use of the antioxidant N-acetyl-L-cysteine (NAC) improved the hPGCLC induction ratio under GK15 conditions, further validating the importance of antioxidant components in PSC-based in vitro experiments.
The H1 human embryonic stem cells (H1-hESCs) used in the current study were purchased from the Chinese Academy of Sciences Stem Cell Culture Collection. All hESC studies were carried out after the approval from the Institutional Review Board of Nanjing Medical University (No. 2019935) and adhered to the Ethical Guidelines for Human Embryonic Stem Cell Research issued by the Ministry of Science and Technology and the National Health Commission of the People's Republic of China as well as the 2021 ISSCR Guidelines for Stem Cell Research and Clinical Translation.
The hPSCs were cultured under the following conditions: 37 ℃, 5% CO2, and saturated humidity. The H1-hESCs were maintained in a feeder-free system using the Essential 8TM medium (Cat. #A1517001, Gibco, Waltham, MA, USA). Cells were plated in six-well plates (Cat. #353046, Corning, NY, USA) pre-coated with Matrigel Matrix (Cat. #354230, Corning, NY, USA) following the manufacturer's protocol. H1-hESCs were used prior to passage 55, while DYR0100-hiPSCs was used before passage 40.
To induce hPGCLC aggregates, the following conditions were used: 37 ℃, 5% CO2, and saturated humidity. As reported previously [12], hPSCs were first induced into human incipient mesoderm-like cells (iMeLCs) and then into hPGCLCs. The hPSCs were treated with TrypLE Select (Cat. #12563-011, Thermo Fisher Scientific, Waltham, MA, USA) to dissociate them into single cells. Then, they were plated at a density of (7.5–8.5)×105 cells per well onto the fibronectin-coated (Cat. # FC010, Millipore, Billerica, MA, USA) six-well plate in either GK15 or N2B27 media supplemented with 50 ng/mL Activin A (Cat. #338-AC-500, R&D Systems, Minneapolis, MN, USA), 100 ng/mL Wnt-3a (Cat. #5036-WN-500, R&D Systems, Minneapolis, MN, USA), and 10 μmol/L of Y-27632 (Cat. #HY-10583, MedChemExpress, Monmouth Junction, NJ, USA). The GK15 medium contained Glasgow's Minimum Essential Medium (GMEM; Cat. #11710035, Thermo Fisher Scientific, Waltham, MA, USA) with 15% KnockOut™ Serum Replacement (KSR; Cat. #10828028, Thermo Fisher Scientific, Waltham, MA, USA), 0.1 mmol/L non-essential amino acid (NEAA; Cat. #11140-050, Thermo Fisher Scientific, Waltham, MA, USA), and 0.1 mmol/L 2-mercaptoethanol (Cat. #21985-023, Thermo Fisher Scientific, Waltham, MA, USA). The N2B27 medium contained Dulbecco's Modified Eagle Medium/Nutrient Mixture F-12 (DMEM-F12; Cat. #11330032, Thermo Fisher Scientific, Waltham, MA, USA) supplemented with N2 (Cat. #17502001, Thermo Fisher Scientific, Waltham, MA, USA), B27 (Cat. #17504044, Thermo Fisher Scientific, Waltham, MA, USA), 0.1 mmol/L NEAA, and 0.1 mmol/L 2-mercaptoethanol.
hPSCs were treated with TrypLE Express (Cat. #12605028, Gibco, Waltham, MA, USA) at 37 ℃ for 3 min, followed by gentle mechanical dissociation using a pipette. After centrifugation, the cell pellets were resuspended in GK15 or N2B27 media, supplemented with 200 ng/mL hBMP4 (Cat. #314-BP, R&D Systems, Minneapolis, MN, USA), 100 ng/mL hSCF (Cat. # BT-SCF, R&D Systems, Minneapolis, MN, USA), 50 ng/mL hEGF (Cat. #236-EG, R&D Systems, Minneapolis, MN, USA), 1,000 U/mL hLIF (Cat. #7734-LF, R&D Systems, Minneapolis, MN, USA), and 10 μmol/L of Y-
Once washed in PBS, the hPGCLC aggregates were dissociated with TrypLE at 37 ℃ for 15 min were dissociated using TrypLE Select. Then EpCAM (1:50, Cat. #369809, BioLegend, San Diego, California, USA) and ITGA6 (1:50, Cat. #313612, BioLegend, San Diego, California, USA) antibodies were incubated with dissociated cells at 37 ℃ for 20 min. Cells expressing high levels of EpCAMhigh and ITGA6high were sorted using the FACS Aria Fusion SOP (San Jose, California, USA). Three replicate experimental results under each condition were included in the statistical analysis.
The samples were fixed with 4% paraformaldehyde in phosphate-buffered saline at room temperature for 20 min, washed three times with Dulbecco’s Phosphate-Buffered Saline (DPBS; Cat. # R21-031-CV, Corning, NY, USA), and permeabilized with 1% Triton X-100 (Cat. #A110694, Sangon, Shanghai, China). After blocking with 5% bovine serum albumin (Cat. # V900933, Sigma-Aldrich, St. Louis, Missouri, USA) in DPBS (with 1% Triton X-100) at room temperature for 4 h, samples were then incubated with a primary antibody diluted in blocking buffer at 4 °C for 24 h. After primary antibody incubation, samples were washed three times with DPBS and incubated with fluorescence-conjugated secondary antibodies diluted in blocking buffer at room temperature for 4 h. Nuclei were stained with Hoechst (Gibco, 62249, 1∶200). Samples were washed three times with DPBS and cleaned for imaging.
The primary antibodies included: goat anti-SOX17 antibody (1∶500, Cat. # AF1924, R&D Systems, Minneapolis, MN, USA;), mouse anti-AP-2 gamma antibody (1∶100, Cat. # SC-12762, Santa Cruz Biotechnology, Dallas, Texas, USA;), and rabbit anti-BLIMP1 antibody (1∶200, Cat. #9115, Cell Signaling Technology, Danvers, MA, USA;). The secondary antibodies (Invitrogen, Waltham, MA, USA) included Hoechst 33342 Nucleic Acid Stains (1∶200, Cat. # H21492), Alexa Fluor 488 donkey anti-mouse antibody (1∶200, Cat. # A-21202), Alexa Fluor 488 donkey anti-rabbit antibody (1∶200, Cat. #A-21206), Alexa Fluor 555 donkey anti-mouse antibody (1:200, Cat. #A-31570), Alexa Fluor 555 donkey anti-rabbit antibody (1∶200, Cat. #A-31572;), and Alexa Fluor 647 donkey anti-goat antibody (1∶200, Cat. #A-21447).
Twenty grams of HistondenzTM (Cat. #D2158, Sigma-Aldrich, St. Louis, Missouri, USA) was dissolved in 15 mL of 0.2× PBS buffer for long-time storage at room temperature.
We took 45 μL of the clearing solution and added it to the CoverWellTM incubation chamber gaskets (Cat. #C18155, Thermo Fisher Scientific, Waltham, MA, USA). Then, we transferred the cleaned samples (after incubation with the secondary antibody) to the CoverWellTM incubation chamber gaskets. After stirring the samples well in the clearing solution using a mouth pipette, we slowly covered the surface of the coverslip from the CoverWellTM incubation chamber gasket side, avoiding foaming as much as possible. The fixed sample was placed in the dark until it was completely cleared.
Brightfield images were taken with an inverted fluorescence microscope TE2000-s (Nikon, Shinagawa, Tokyo, Japan) equipped with an AxioCam HRc camera (Zeiss, Oberkochen, Baden-Württemberg, Germany) and another inverted microscope, Eclipse Ti2-U (Nikon).
Confocal immunofluorescence images of hPGCLC aggregates were acquired using Zeiss LSM 800 confocal microscopes. Images were processed using ZEN (Zeiss).
Cells were seeded at a density of 1.5 × 106 cells per well under hPGCLC induction conditions (including GK15 and N2B27 induction conditions) in six replicates. Aggregates were collected at 24-h intervals for flow cytometry analysis. At each time point, cells were digested and counted using the Thermo Fisher Life Countess II Automated Cell Counter (Waltham, MA, USA).
Once washed in PBS, the hPGCLC aggregates were dissociated with TrypLE at 37 ℃ for 15 min. Then, both 20 nmol/L Mitotracker Green (MTG, Cat. # M7514, Thermo Fisher Scientific, Waltham, MA, USA) and 20 nmol/L Tetramethylrhodamine Methyl Ester (TMRM, Cat. # I34361, Thermo Fisher Scientific, Waltham, MA, USA) were incubated with the dissociated cells at 37 ℃ for 30 min. After incubation, the cells were washed three times with DPBS to remove excess dye. Cells stained with MTG and TMRM were analyzed using the FACS Aria Fusion SOP. Three replicate experimental results under each condition were included in the statistical analysis.
A Reactive Oxygen Species (ROS) Assay kit (Cat. #50101ES01, Yeasen, Shanghai, China) was used for this experiment. Once washed in PBS, the hPGCLC aggregates were dissociated with TrypLE at 37 ℃ for 15 min. The appropriately diluted probe (10 μmol/L) was added and incubated with the cells in a 37 ℃ cell incubator for 30 min. The samples were mixed gently every 3–5 min to ensure thorough contact between the probe and the cells. After incubation, the cells were washed three times with DPBS to remove excess dye. The incubated cells were analyzed using the FACS Aria Fusion SOP.
The antioxidant NAC (MedChemExpress, HY-B0215) was set in groups of 0, 0.25, 0.5, 0.75, and 1.0 mmol/L. NAC was added continuously during the hPGCLC induction process in GK15 conditions, and relevant analyses were performed four days after sampling.
Total RNA was extracted from human PGCLC induction from H1-ESCs using the FreeZol Reagent (Cat. # R711-02, Vazyme, Nanjing, China), following the manufacturer’s protocol. For transcriptome sequencing, 1 μg of RNA per sample was used as input material. Sequencing libraries were prepared using the NEBNext® Ultra™ RNA Library Prep Kit for Illumina® (NEB, USA) according to the manufacturer’s protocol. Index codes were incorporated into each sample to facilitate sequence identification. The clustering of index-coded libraries was performed on a cBot Cluster Generation System using the TruSeq PE Cluster Kit v3-cBot-HS (Illumina, USA) according to the manufacturer’s instructions. Following cluster generation, the libraries were sequenced on an Illumina NovaSeq platform, generating 150 bp paired-end reads. Sequencing data quality was assessed using FastQC (v0.11.5) to ensure adequate read quality, and adapter contamination levels were monitored.
The paired-end clean reads were aligned to the human reference genome (GRCh38, GENCODE annotation release v34) using HISAT2 (version 2.1.0)[20] with default parameters. The gene-level read counts were quantified using featureCounts (version 2.0.0)[21] with the GTF annotation file from GENCODE v34. Only uniquely mapped reads were included in the downstream analysis to ensure the accuracy of gene expression estimates.
Transcript abundance was calculated as TPM (transcripts per million), and all subsequent analyses were based on log2(TPM + 1)-transformed values. Differential expression analysis was performed using the Deseq2 package (version 1.36.0)[22] within the R software environment (version 3.6.3). Genes were considered differentially expressed if they met the thresholds of |log2(fold change)| > 1 and adjusted P-value < 0.05, as calculated using the Benjamini-Hochberg method to control for false discovery rates.
To visualize data variability and sample clustering, principal component analysis (PCA) was performed using the prcomp function in R. Heatmaps of differentially expressed genes were generated using the ComplexHeatmap package (version 2.15.3)[23], and other visualizations were constructed using ggpubr (version 0.4.0) and tidyverse (version 1.3.0). For additional 3D visualizations of expression patterns, the rgl package (version 0.100.54) was employed.
Functional enrichment analyses, including Gene Ontology (GO) and Kyoto Encyclopedia of Genes and Genomes (KEGG) pathway analyses, were conducted using the DAVID bioinformatics web tool[24]. Results of GO and KEGG enrichment analyses were filtered using a significance threshold of P-value < 0.05.
The public datasets utilized in this study are available from the Gene Expression Omnibus (GEO) database under accession numbers GSE60138, GSE67259, and GSE86146. The data generated in this study will be made publicly available in a suitable repository upon acceptance of the manuscript.
Student's t-tests were used for comparisons between two groups. These analyses were performed using GraphPad Prism version 8.0 software. All data points collected in the current study were included in statistical analyses. All data were presented as mean ± standard error of the mean, and a P-value of less than 0.05 was considered to indicate statistical significance.
In recent years, hPSCs have been used to induce hPGCLCs in vitro via a two-step approach with different basal media (aRB27 and GK15) containing different cytokines [11, 12]. Building on these published methods, we assessed ways to optimize and develop more effective hPGCLC induction protocols in vitro. We performed tests with four combinations of basal media, including the traditional GK15 medium (GMEM and 15% KSR) for inducing hPGCLCs and the optimized N2B27 medium (DMEM/F12, N2, and B27) that has not been previously used for the hPGCLC induction (Supplementary Fig. 1A, available online).
First, hPSCs were pre-induced into iMeLCs for 42 h in the N2B27 medium supplemented with WNT3A and activin A. Then, iMeLCs were further induced into PGCLCs in the N2B27 medium containing BMP4, SCF, LIF, EGF, and a ROCK inhibitor under floating aggregate conditions (Fig. 1A). Bright-field imaging revealed that the aggregates exhibited the largest volume under combination 1, followed by combination 2 (Supplementary Fig. 1B). Using FCM analysis with the known surface markers (EpCAM and ITGA6) of PGCLCs on day 4 of the aggregate induction, we found that the PGCLC induction efficiency was greater under combination 1, followed by combination 2 (Supplementary Fig. 1B). Therefore, we performed further tests using the N2B27 medium for inducing both iMELCs and hPGCLCs, with GK15 medium used as the control.
Bright-field imaging on day 1 showed that aggregates was larger in the N2B27 medium than in the GK15 medium, indicating that iMeLCs cultured under these conditions had a greater antiapoptotic capacity. Aggregates in N2B27 showed more noticeable proliferation throughout the induction process (Fig. 1B). The number of proliferating aggregates in GK15 and N2B27 media during induction also supported this observation (Supplementary Fig. 1C). Through FCM analysis of aggregates from days 1 to 4, we found that the PGCLC induction efficiency was greater under N2B27 than in GK 15 culture conditions (Fig. 1C and 1D). A distinct EpCAM+ and ITGA6+ population appeared as early as day 2 under N2B27 culture conditions, whereas population clustering was not observed until day 3 under GK15 culture conditions (Fig. 1C).
To confirm that N2B27 culture conditions are more conducive to PGCLC induction, we performed immunofluorescence staining on the day 4 aggregates using known PGC markers (i.e., SOX17, BLIMP1, and TFAP2C). Under N2B27 culture conditions, there were more colocalizing cells of SOX17, BLIMP1, and TFAP2C scattered throughout the aggregates. In contrast, under GK15 culture conditions, these colocalized cells were more concentrated in the outer layer rather than in the center of the aggregates (Fig. 1E).
To further characterize the induced hPGCLCs, we sorted EpCAM+ and ITGA6+ cells using FACS, performed bulk RNA-seq, and compared these results with the published data[11, 12]. We performed Pearson correlation analysis of the differentially expressed genes (DEGs) among all the cell types. The clustering analysis further supported that the EpCAM/ITGA6 double-positive cells had the greatest correlation with d4 PGCLC from the published data (Fig. 1F). PCA results further confirmed this consistency (Supplementary Fig. 1D). Among the relevant cell types from the two-step induction process, the EpCAM/ITGA6 double-positive cells presented the fewest DEGs [log2(TPM + 1) > 4 and log2(fold change) > 2], compared with the BLIMP1-2A-tdTomato and TFAP2C-2A-EGFP double-positive cells on day 4 (d4BTAG+) in both culture media (Supplementary Fig. 1E and Supplementary Table 1). The expression levels of early PGC markers (SOX17, TFAP2C, and BLIMP1) and germ layer-associated genes (EOMES, GATA4, and GATA6) were comparable between the EpCAM/ITGA6 double-positive cells and the d4BTAG+ cells. However, in the EpCAM/ITGA6 double-positive cells, the expression levels of pluripotency-related genes (NANOS3, NANOG, and POU5F1) were expressed at higher levels (Supplementary Fig. 1F). These findings suggested that the hPGCLCs derived under these conditions exhibited robust pluripotency, resembling a state of naïve pluripotency to some extent. These observations were consistent with previous reports[25, 26].
To further evaluate the agreement between the in vitro-induced hPGCLCs and the in vivo developmental processes, we compared hPGCLCs derived from both culture media with single-cell transcriptomic profiles of fetal germ cells (FGCs) throughout embryonic development [27]. The PCA results revealed a directional and progressive transition in cellular properties during the development of FGCs with different characteristics. Notably, hPGCLCs presented a greater similarity to the cell cluster containing FGC-1 females and FGC-1/2 males (Fig. 1G). In contrast, the differences between hPGCLCs derived from N2B27 and those derived from GK15 were negligible. To determine which embryonic stage of FGCs1/2 was more similar to that of hPGCLCs derived from the GK15 and N2B27 conditions, we conducted a similarity analysis. The unsupervised hierarchical clustering revealed that our hPGCLCs were most similar to male FGC-1 cells from four-week-old embryos (Supplementary Fig. 1G). PCA showed similar results (Supplementary Fig. 1H).
These findings suggested that the cultivated cells with PGC characteristics and the EpCAM/ITGA6 double-positive cells during induction could be defined as hPGCLCs, with a relatively high induction efficiency achieved under N2B27 culture conditions.
We focused on the dynamics of hPGCLCs derived from two induction conditions. FCM was performed every 8 h during the hPGCLC induction under GK15 and N2B27 conditions via the PGC surface markers ITGA6 and EpCAM. EpCAM and ITGA6 have previously been reported to be expressed on hPSCs, PGCLCs, and other cell types[28]. Under N2B27 induction conditions, the EpCAM/ITGA6 double-positive cell population, representing hPGCLCs, emerged between 40 h and 48 h, at which point three distinct cell types were identified (Fig. 2A and 2C). Under GK15 induction conditions, the appearance of the same cell population was delayed, occurring at about 48–64 h. During the subsequent period, the proportion of EpCAM/ITGA6 double-positive (+/+) cells progressively increased, with a more prominent separation from other cell populations (Fig. 2B and 2C). The differentiation process was described as the transition of the EpCAM-positive/ITGA6-weakly positive (+/−) cells to the EpCAM/ITGA6 double-negative (−/−) and the EpCAM/ITGA6 double-positive (+/+) cells.
We detected transcriptomic differences among the three cell populations under N2B27 and GK15 conditions. The differences between EpCAM-positive/ITGA6-weakly positive (+/−) cells in the two media were minimal, whereas significant differences were found among the double-negative (−/−) cells (Fig. 2D). These results suggest that in aggregates, there is considerable uncertainty in the differentiation of cell types other than PGCLCs, and these cells may influence the generation of PGCLCs.
We separately analyzed the differences between the three cell types derived from the GK15 and N2B27 conditions and merged them to obtain 847 genes. The findings indicated that these genes were considerably different in the three cell types regardless of the medium. The unsupervised hierarchical clustering classified such genes into six clusters, with each cluster showing specific expression in distinct cell types. The Cluster 1 and 2 genes (118 and 226 genes, respectively), especially the Cluster 2 genes with relatively high expression levels, were specifically expressed in the EpCAM/ITGA6 double-positive cells and enriched with GO terms, such as “stem cell population maintenance”, “male sex differentiation”, and “developmental process involved in reproduction”. In contrast, the Cluster 5 genes (64 genes) exhibited specific enriched expression in the EpCAM/ITGA6 double-negative cells and were enriched with GO terms related to blood and blood vessels, such as “vasculature development”. Consistent with previous results, Clusters 3 and 4 genes (279 and 123 genes, respectively) were enriched with GO terms correlated with epithelial characteristics and cell adhesion and migration. Cluster 6 genes (37 genes) presented the common characteristics of double-negative cells and were enriched with GO terms such as “neurogenesis” (Fig. 2E and Supplementary Table 2). These findings suggest that within aggregates, the EpCAM-positive/ITGA6-weakly positive (+/−) and the EpCAM/ITGA6 double-negative (−/−) cell populations may be correlated with the development of the hematopoietic system, neural cells, or epithelial cells, resembling the multilineage cell fate observed in undirected induced embryoid bodies[29]. Stem cells exhibit plasticity and uncertainty in response to various cytokines. Under N2B27 induction conditions, hPGCLCs were more readily differentiated within the aggregates. In contrast, under GK15 induction conditions, even with the same cytokines and concentrations, differentiation was skewed toward non-hPGCLC cell types.
These results showed that under N2B27 induction conditions, hPGCLCs were generated earlier, ensuring that hPSCs differentiated into hPGCLCs rather than other cell types that also responded to LIF, EGF, SCF, and BMP4.
We focused on the main reasons for the induction of hPGCLCs under two different culture conditions. To identify the global gene expression characteristics of hPGCLCs derived from the two media, their transcriptome data were compared. Among the genes whose expression was upregulated in hPGCLCs cultured in the N2B27 medium, most genes, such as PDH, HIF1A, SLC2A1, PHD2, and DNIP3, are involved primarily in the cellular hypoxic response system, regulating the hypoxia-inducible factor (HIF) pathway (Fig. 3A and Supplementary Table 3), which helps cells adapt to hypoxic or oxidative stress environment[30-32]. Both GO and KEGG analyses were subsequently performed on the DEGs. The results revealed that significant biological terms, such as fructose and mannose metabolism, the HIF-1 signaling pathway, and the response to oxygen levels, were enriched under N2B27 culture conditions (Fig. 3B, 3C and Supplementary Table 3). These results suggested that under N2B27 induction conditions, PGCLCs responded more actively to the in vitro environment, mitigating potential negative effects on development. This may be because the N2B27 culture environment is rich in antioxidants from the DMEM-F12 medium and B27 supplements, whereas the GK15 culture environment lacks these components to a similar extent. Under the GK15 condition, PSCs appear inadequately equipped to manage the hypoxic environment, resulting in oxidative stress and mitochondrial dysfunction.
Studies have indicated that during the induction of PGCLCs from PSCs, energy production depends not only on glycolysis but also requires oxidative phosphorylation, necessitating mitochondrial activation[33-35]. To investigate mitochondrial activity differences in aggregates under the two culture conditions, MTG was used to indicate the total mitochondrial pool, while TMRM labeled active mitochondria with intact membranes. The MTG and TMRM-labeled cells were analyzed through FCM. A higher proportion of TMRM-positive cells was observed under the N2B27 condition (Fig. 3D and 3E). Furthermore, TMRM-positive cells from N2B27 induction conditions exhibited a generally higher mitochondrial membrane potential (MMP), indicating that N2B27 conditions provide a better protection for mitochondrial homeostasis (Fig. 3F). This observation suggests that the induction process under N2B27 conditions successfully transitions to oxidative phosphorylation for energy production, whereas energy production under the GK15 condition remains relatively insufficient, adversely affecting the PGCLC generation efficiency.
In addition, ROS levels were examined, as their accumulation occurs not only under hypoxic conditions but also as a result of mitochondrial dysfunction[36, 37]. ROS levels were compared between aggregates derived from different induction conditions, revealing significantly lower ROS levels under N2B27 than GK15 induction conditions (Fig. 3G).
The above results indicated that the aggregates under GK15 and N2B27 induction conditions responded differently to hypoxia during induction, which may be due to the presence of more antioxidants under N2B27 induction conditions (Fig. 3H).
The experimental results showed that antioxidants might enhance the in vitro induction of PGCLCs. To further investigate this phenomenon, we added antioxidants under GK15 induction conditions to evaluate their ability to improve the PGCLC induction efficiency. We identified NAC, an antioxidant that targets the HIF-1 pathway, which can reduce oxidative stress and enhance mitochondrial bioenergetics[38, 39].
The experiments included a control group (0 mmol/L), 0.25 mmol/L-NAC group, 0.5 mmol/L-NAC group, 0.75 mmol/L-NAC group, and 1.0 mmol/L-NAC group. The NAC addition started on day 0 of the PGCLC induction process, and the samples were collected for analysis on day 4 of culture (Fig. 4A). Adding NAC did not significantly affect the aggregate volume (Fig. 4B). However, the FCM analysis results revealed a considerable change in the proportion of the EpCAM and ITGA6 double-positive cells, with a particularly pronounced effect at a concentration of 0.5 mmol/L, where the proportion doubled compared with other groups (Fig. 4C). Next, we performed immunofluorescence staining on day 4 aggregates to assess the expression of SOX17, TFAP2C, and BLIMP1, and the results revealed that the proportion of triple-positive cells increased with increasing concentrations of NAC (Fig. 4D). In addition, ROS levels were compared between aggregates derived from different induction conditions, revealing significantly lower ROS levels after the addition of NAC under GK15 induction conditions than GK15 induction alone (Fig. 4E).
In the 0.5 mmol/L NAC group, EpCAM+ and ITGA6+ cells were sorted via FACS. Then, bulk RNA-seq was performed, and these data were compared with the PGCLC data obtained under GK15 induction conditions and with published data. The Pearson correlation analysis of DEGs across all cell types revealed that the EpCAM and ITGA6 double-positive cells under GK15 induction conditions supplemented with 0.5 mmol/L NAC presented the highest correlation with GK15-induced hPGCLCs, which was also highly correlated with previously published hPGCLC data (Fig. 4F). To further investigate the transcriptomic differences between hPGCLCs induced with or without NAC in GK15 medium, we detected almost no DEGs. The P-value distribution was nearly uniform, with only a few low P-values (Supplementary Fig. 2A), suggesting that there were no statistically significant differences in gene expression between the two conditions (GK15 induction conditions with or without 0.5 mmol/L NAC). This inference was further supported by the expression data, where the distributions of gene expression levels were similar between the two conditions (Supplementary Fig. 2B), with no apparent shift or clustering that would indicate differential expression. We were also interested in the transcriptional similarity between the GK15-induced hPGCLCs supplied with 0.5 mmol/L NAC and the N2B27-induced PGCLCs. To compare the overall differences between the two groups, we performed batch correction on the data from the two batches and then conducted a similarity analysis. The results showed that the GK15-induced hPGCLCs supplied with NAC groups clustered together with the GK15-induced hPGCLC groups (from both batches), while the N2B27-induced hPGCLC groups formed a distinct cluster (Supplementary Fig. 2C).
These results indicated that an optimal concentration of NAC effectively increased the in vitro induction efficiency of hPGCLCs, highlighting that those antioxidants play an important role in hPGCLC induction.
We developed a new hPGCLC induction system in which the N2B27 medium enriched with antioxidant components was used to induce hPGCLCs. The current study revealed that the N2B27 medium enhanced cell survival in an in vitro environment by optimizing oxidative stress responses, improving mitochondrial function, and reducing ROS accumulation, thereby increasing the induction efficiency of PGCLCs. Furthermore, the addition of the antioxidant NAC further validated the crucial role of antioxidant components in PGCLC induction, providing a new strategy and approach for the in vitro induction of hPGCLCs.
The induction of germ cell meiosis in vitro is a longstanding goal for addressing azoospermia, and this area of research has received significant attention over the years[40, 41]. Regardless of the specific methodology used, the generation of hPGCLCs represents an indispensable initial step. However, achieving efficient and large-scale production of hPGCLCs in vitro remains a significant challenge. The development of PGCs in vivo occurs under unique conditions within the early embryonic environment. This process is governed by intrinsic regulatory mechanisms that determine cell fate, along with interactions between the embryonic microenvironment and neighboring embryonic cells[42-44]. Thus, compared with conventional two-dimensional adherent culture systems, three-dimensional aggregate systems, such as those resembling embryoid bodies, provide a more favorable environment for inducing PGCs[45]. These systems recapitulate the microenvironment of early embryonic development more accurately, enhancing the subsequent generation of functional spermatogonia-like cells, spermatocyte-like cells, and even sperm-like cells.
In stem cell experiments, the in vitro microenvironment consistently presents challenges for stem cell research and applications. Key factors such as temperature, humidity, oxygen concentration, and pressure are critical, with oxygen concentration being particularly important[46, 47]. Studies have shown that PSCs exhibit enhanced stability under hypoxic conditions[15-17]. However, the optimal oxygen concentration may vary depending on the specific experimental application of PSCs. For example, during the generation of PGCs, the process is not solely dependent on glycolysis; oxidative phosphorylation also plays a crucial role[33-35]. Therefore, determining this optimal concentration requires comprehensive testing to minimize oxidative stress and ROS accumulation, which can disrupt energy production and metabolism in cells, ultimately compromising experimental outcomes. In cases where oxidative stress is detected, the controlled addition of antioxidants can mitigate adverse effects, helping cells adapt to oxidative stress and attain homeostasis. This approach ensures the maintenance of cell function and improves the reliability of experimental results.
NAC is a common antioxidant that generally exerts its antioxidant effects by increasing intracellular levels of glutathione. As a precursor to glutathione, NAC is converted into cysteine in the body, which in turn promotes glutathione synthesis. In this way, NAC helps reduce oxidative stress and has demonstrated broad therapeutic potential in the treatment of oxidative stress-related diseases, liver toxicity, poisoning, respiratory diseases, as well as certain neurological and psychiatric disorders[48]. The addition of NAC during the hPGCLC induction process can effectively enhance the induction efficiency of hPGCLCs and reduce ROS levels in the culture environment, which is consistent with the results obtained in the N2B27 medium enriched with antioxidants. Therefore, antioxidants play a crucial role in the induction of hPGCLCs. Looking ahead, NAC’s antioxidant properties position it as a promising candidate for future clinical applications in reproductive medicine. Given that oxidative stress is a key factor contributing to age-related infertility and idiopathic reproductive disorders, NAC supplementation may help protect germ cells from oxidative damage, preserve oocyte and sperm quality, and improve assisted reproductive technologies (ART) outcomes. Moreover, its ability to modulate the cellular redox state could be beneficial in delaying age-related decline in fertility and supporting gonadal function in individuals undergoing chemotherapy or other gonadotoxic treatments. Further research is warranted to explore the long-term effects of NAC on human germ cell maintenance, gametogenesis, and overall reproductive health.
Although the current study describes ways to improve the induction rate of PGCLCs under GK15 induction conditions via the antioxidant NAC, the efficiency of hPGCLC induction in vitro can be further increased. Future studies may focus on the metabolic pathways involved in PGC development or the cell-cell interactions during early embryonic development. By recapitulating the in vivo environment more accurately, the production efficiency and functionality of hPGCLCs may be further improved.
We thank the Jiang Min research group from Westlake University for providing the mitochondrial dye.
This research was funded by the National Key R&D Program grants 2022YFC2702800 (Y.Y.) and 2021YFC2700302 (Y.Y.), as well as the National Natural Science Foundation of China grants 82122025 (Y.Y.), 82221005 (J.S.), and 82201763 (L.L.).
CLC number: R321.1, Document code: A
The authors reported no conflict of interests.
[1] |
Cheng H, Shang D, Zhou R. Germline stem cells in human[J]. Signal Transduct Target Ther, 2022, 7(1): 345. doi: 10.1038/s41392-022-01197-3
|
[2] |
Saitou M, Yamaji M. Primordial germ cells in mice[J]. Cold Spring Harb Perspect Biol, 2012, 4(11): a008375. https://cshperspectives.cshlp.org/content/4/11/a008375.long
|
[3] |
Ohinata Y, Ohta H, Shigeta M, et al. A signaling principle for the specification of the germ cell lineage in mice[J]. Cell, 2009, 137(3): 571–584. doi: 10.1016/j.cell.2009.03.014
|
[4] |
Chen D, Sun N, Hou L, et al. Human primordial germ cells are specified from lineage-primed progenitors[J]. Cell Rep, 2019, 29(13): 4568–4582. e5.
|
[5] |
Nikolic A, Volarevic V, Armstrong L, et al. Primordial germ cells: current knowledge and perspectives[J]. Stem Cells Int, 2016, 2016(1): 1741072. doi: 10.1155/2016/1741072
|
[6] |
Ishii T. Human iPS cell-derived germ cells: current status and clinical potential[J]. J Clin Med, 2014, 3(4): 1064–1083. doi: 10.3390/jcm3041064
|
[7] |
Ishikura Y, Yabuta Y, Ohta H, et al. In vitro derivation and propagation of spermatogonial stem cell activity from mouse pluripotent stem cells[J]. Cell Rep, 2016, 17(10): 2789–2804. doi: 10.1016/j.celrep.2016.11.026
|
[8] |
Zhou Q, Wang M, Yuan Y, et al. Complete meiosis from embryonic stem cell-derived germ cells in vitro[J]. Cell Stem Cell, 2016, 18(3): 330–340. doi: 10.1016/j.stem.2016.01.017
|
[9] |
Yao C, Yao R, Luo H, et al. Germline specification from pluripotent stem cells[J]. Stem Cell Res Ther, 2022, 13(1): 74. doi: 10.1186/s13287-022-02750-1
|
[10] |
Yuan Y, Zhou Q, Wan H, et al. Generation of fertile offspring from Kit W/Kit WV mice through differentiation of gene corrected nuclear transfer embryonic stem cells[J]. Cell Res, 2015, 25(7): 851–863. doi: 10.1038/cr.2015.74
|
[11] |
Irie N, Weinberger L, Tang WWC, et al. SOX17 is a critical specifier of human primordial germ cell fate[J]. Cell, 2015, 160(1-2): 253–268. doi: 10.1016/j.cell.2014.12.013
|
[12] |
Sasaki K, Yokobayashi S, Nakamura T, et al. Robust in vitro induction of human germ cell fate from pluripotent stem cells[J]. Cell Stem Cell, 2015, 17(2): 178–194. doi: 10.1016/j.stem.2015.06.014
|
[13] |
Sugawa F, Araúzo‐Bravo MJ, Yoon J, et al. Human primordial germ cell commitment in vitro associates with a unique PRDM14 expression profile[J]. EMBO J, 2015, 34(8): 1009–1024. doi: 10.15252/embj.201488049
|
[14] |
Mohyeldin A, Garzón-Muvdi T, Quiñones-Hinojosa A. Oxygen in stem cell biology: a critical component of the stem cell niche[J]. Cell Stem Cell, 2010, 7(2): 150–161. doi: 10.1016/j.stem.2010.07.007
|
[15] |
Keith B, Simon MC. Hypoxia-inducible factors, stem cells, and cancer[J]. Cell, 2007, 129(3): 465–472. doi: 10.1016/j.cell.2007.04.019
|
[16] |
Ezashi T, Das P, Roberts RM. Low O2 tensions and the prevention of differentiation of hES cells[J]. Proc Natl Acad Sci U S A, 2005, 102(13): 4783–4788. doi: 10.1073/pnas.0501283102
|
[17] |
Prasad SM, Czepiel M, Cetinkaya C, et al. Continuous hypoxic culturing maintains activation of notch and allows long‐term propagation of human embryonic stem cells without spontaneous differentiation[J]. Cell Prolif, 2009, 42(1): 63–74. doi: 10.1111/j.1365-2184.2008.00571.x
|
[18] |
Zachar V, Prasad SM, Weli SC, et al. The effect of human embryonic stem cells (hESCs) long-term normoxic and hypoxic cultures on the maintenance of pluripotency[J]. In Vitro Cell Dev Biol Anim, 2010, 46(3-4): 276–283. doi: 10.1007/s11626-010-9305-3
|
[19] |
Chaudhari P, Ye Z, Jang YY. Roles of reactive oxygen species in the fate of stem cells[J]. Antioxid Redox Signal, 2014, 20(12): 1881–1890. doi: 10.1089/ars.2012.4963
|
[20] |
Kim D, Langmead B, Salzberg SL. HISAT: a fast spliced aligner with low memory requirements[J]. Nat Methods, 2015, 12(4): 357–360. doi: 10.1038/nmeth.3317
|
[21] |
Liao Y, Smyth GK, Shi W. featureCounts: an efficient general purpose program for assigning sequence reads to genomic features[J]. Bioinformatics, 2014, 30(7): 923–930. doi: 10.1093/bioinformatics/btt656
|
[22] |
Love MI, Huber W, Anders S. Moderated estimation of fold change and dispersion for RNA-seq data with DESeq2[J]. Genome Biol, 2014, 15(12): 550. doi: 10.1186/s13059-014-0550-8
|
[23] |
Gu Z, Eils R, Schlesner M. Complex heatmaps reveal patterns and correlations in multidimensional genomic data[J]. Bioinformatics, 2016, 32(18): 2847–2849. doi: 10.1093/bioinformatics/btw313
|
[24] |
Sherman BT, Hao M, Qiu J, et al. DAVID: a web server for functional enrichment analysis and functional annotation of gene lists (2021 update)[J]. Nucleic Acids Res, 2022, 50(W1): W216–W221. doi: 10.1093/nar/gkac194
|
[25] |
Chen D, Liu W, Zimmerman J, et al. The TFAP2C-regulated OCT4 naive enhancer is involved in human germline formation[J]. Cell Rep, 2018, 25(13): 3591–3602. e5.
|
[26] |
Yu S, Zhou C, He J, et al. BMP4 drives primed to naïve transition through PGC-like state[J]. Nat Commun, 2022, 13(1): 2756. doi: 10.1038/s41467-022-30325-4
|
[27] |
Li L, Dong J, Yan L, et al. Single-cell RNA-seq analysis maps development of human germline cells and gonadal niche interactions[J]. Cell Stem Cell, 2017, 20(6): 858–873. e4.
|
[28] |
Vijayakumar S, Sala R, Kang G, et al. Monolayer platform to generate and purify primordial germ-like cells in vitro provides insights into human germline specification[J]. Nat Commun, 2023, 14(1): 5690. doi: 10.1038/s41467-023-41302-w
|
[29] |
Brickman JM, Serup P. Properties of embryoid bodies[J]. Wiley Interdiscip Rev Dev Biol, 2017, 6(2): e259. doi: 10.1002/wdev.259
|
[30] |
Majmundar AJ, Wong WJ, Simon MC. Hypoxia-inducible factors and the response to hypoxic stress[J]. Mol Cell, 2010, 40(2): 294–309. doi: 10.1016/j.molcel.2010.09.022
|
[31] |
Zepeda AB, Pessoa Jr A, Castillo RL, et al. Cellular and molecular mechanisms in the hypoxic tissue: role of HIF‐1 and ROS[J]. Cell Biochem Funct, 2013, 31(6): 451–459. doi: 10.1002/cbf.2985
|
[32] |
Solaini G, Baracca A, Lenaz G, et al. Hypoxia and mitochondrial oxidative metabolism[J]. Biochim Biophys Acta Bioenerg, 2010, 1797(6-7): 1171–1177. doi: 10.1016/j.bbabio.2010.02.011
|
[33] |
Bothun AM, Woods DC. Inherent mitochondrial activity influences specification of the germ line in pluripotent stem cells[J]. Heliyon, 2020, 6(4): e03651. doi: 10.1016/j.heliyon.2020.e03651
|
[34] |
Tischler J, Gruhn WH, Reid J, et al. Metabolic regulation of pluripotency and germ cell fate through α‐ketoglutarate[J]. EMBO J, 2019, 38(1): e99518. doi: 10.15252/embj.201899518
|
[35] |
Lu V, Teitell MA. Alpha‐ketoglutarate: a “magic” metabolite in early germ cell development[J]. EMBO J, 2019, 38(1): e100615. doi: 10.15252/embj.2018100615
|
[36] |
Zorov DB, Juhaszova M, Sollott SJ. Mitochondrial ROS-induced ROS release: an update and review[J]. Biochim Biophys Acta Bioenerg, 2006, 1757(5-6): 509–517. doi: 10.1016/j.bbabio.2006.04.029
|
[37] |
Averill-Bates D. Reactive oxygen species and cell signaling. Review[J]. Biochim Biophys Acta Mol Cell Res, 2024, 1871(2): 119573. doi: 10.1016/j.bbamcr.2023.119573
|
[38] |
Kumar P, Osahon OW, Sekhar RV. GlyNAC (glycine and N-acetylcysteine) supplementation in mice increases length of life by correcting glutathione deficiency, oxidative stress, mitochondrial dysfunction, abnormalities in mitophagy and nutrient sensing, and genomic damage[J]. Nutrients, 2022, 14(5): 1114. doi: 10.3390/nu14051114
|
[39] |
Dhouib IE, Jallouli M, Annabi A, et al. A minireview on N-acetylcysteine: an old drug with new approaches[J]. Life Sci, 2016, 151: 359–363. doi: 10.1016/j.lfs.2016.03.003
|
[40] |
Ishikura Y, Ohta H, Sato T, et al. In vitro reconstitution of the whole male germ-cell development from mouse pluripotent stem cells[J]. Cell Stem Cell, 2021, 28(12): 2167–2179. e9.
|
[41] |
Murase Y, Yabuta Y, Ohta H, et al. Long‐term expansion with germline potential of human primordial germ cell‐like cells in vitro[J]. EMBO J, 2020, 39(21): e104929. doi: 10.15252/embj.2020104929
|
[42] |
Saitou M, Yamaji M. Germ cell specification in mice: signaling, transcription regulation, and epigenetic consequences[J]. Reproduction, 2010, 139(6): 931–942. doi: 10.1530/REP-10-0043
|
[43] |
Sasaki K, Nakamura T, Okamoto I, et al. The germ cell fate of cynomolgus monkeys is specified in the nascent amnion[J]. Dev Cell, 2016, 39(2): 169–185. doi: 10.1016/j.devcel.2016.09.007
|
[44] |
Saitou M. Germ cell specification in mice[J]. Curr Opin Genet Dev, 2009, 19(4): 386–395. doi: 10.1016/j.gde.2009.06.003
|
[45] |
Keller GM. In vitro differentiation of embryonic stem cells[J]. Curr Opin Cell Biol, 1995, 7(6): 862–869. doi: 10.1016/0955-0674(95)80071-9
|
[46] |
Jež M, Rožman P, Ivanović Z, et al. Concise review: the role of oxygen in hematopoietic stem cell physiology[J]. J Cell Physiol, 2015, 230(9): 1999–2005. doi: 10.1002/jcp.24953
|
[47] |
Samal JRK, Rangasami VK, Samanta S, et al. Discrepancies on the role of oxygen gradient and culture condition on mesenchymal stem cell fate[J]. Adv Healthc Mater, 2021, 10(6): 2002058. doi: 10.1002/adhm.202002058
|
[48] |
Dodd S, Dean O, Copolov DL, et al. N-acetylcysteine for antioxidant therapy: pharmacology and clinical utility[J]. Expert Opin Biol Ther, 2008, 8(12): 1955–1962. doi: 10.1517/14728220802517901
|
[1] | Natalia V. Naryzhnaya, Leonid N. Maslov, Ivan A. Derkachev, Huijie Ma, Yi Zhang, N. Rajendra Prasad, Nirmal Singh, Feng Fu, Jianming Pei, Akpay Sarybaev, Akylbek Sydykov. The effect of an adaptation to hypoxia on cardiac tolerance to ischemia/reperfusion[J]. The Journal of Biomedical Research, 2023, 37(4): 230-254. DOI: 10.7555/JBR.36.20220125 |
[2] | Xing Ming, Wang Na, Zeng Hanyi, Zhang Jun. α-ketoglutarate promotes the specialization of primordial germ cell-like cells through regulating epigenetic reprogramming[J]. The Journal of Biomedical Research, 2021, 35(1): 36-46. DOI: 10.7555/JBR.34.20190160 |
[3] | Zhang Zhijia, Hou Yuxing, Li Jiantao, Tang Chao, Que Linli, Tan Qian, Li Yuehua. TIR/BB-loop mimetic AS-1 protects vascular endothelial cells from injury induced by hypoxia/reoxygenation[J]. The Journal of Biomedical Research, 2020, 34(5): 343-350. DOI: 10.7555/JBR.33.20190030 |
[4] | Tiwari-Heckler Shilpa, Jiang Z. Gordon, Popov Yury, J. Mukamal Kenneth. Daily high-dose aspirin does not lower APRI in the Aspirin-Myocardial Infarction Study[J]. The Journal of Biomedical Research, 2020, 34(2): 139-142. DOI: 10.7555/JBR.33.20190041 |
[5] | Minbo Zang, Qiao Zhou, Yunfei Zhu, Mingxi Liu, Zuomin Zhou. Effects of chemotherapeutic agent bendamustine for nonhodgkin lymphoma on spermatogenesis in mice[J]. The Journal of Biomedical Research, 2018, 32(6): 442-453. DOI: 10.7555/JBR.31.20170023 |
[6] | Kaibo Lin, Shikun Zhang, Jieli Chen, Ding Yang, Mengyi Zhu, Eugene Yujun Xu. Generation and functional characterization of a conditional Pumilio2 null allele[J]. The Journal of Biomedical Research, 2018, 32(6): 434-441. DOI: 10.7555/JBR.32.20170117 |
[7] | Huanqiang Wang, Congying Yang, Siyuan Wang, Tian Wang, Jingling Han, Kai Wei, Fucun Liu, Jida Xu, Xianzhen Peng, Jianming Wang. Cell-free plasma hypermethylated CASZ1, CDH13 and ING2 are promising biomarkers of esophageal cancer[J]. The Journal of Biomedical Research, 2018, 32(6): 424-433. DOI: 10.7555/JBR.32.20170065 |
[8] | Qun Zhang, Xiaofeng Zhang, Mengmeng Zhao, Hanpeng Huang, Ning Ding, Xilong Zhang, Hong Wang. Correlation of obstructive sleep apnea hypopnea syndrome with metabolic syndrome in snorers[J]. The Journal of Biomedical Research, 2014, 28(3): 222-227. DOI: 10.7555/JBR.28.20120120 |
[9] | Xiangrong Zuo, Feng Zong, Hui Wang, Qiang Wang, Weiping Xie, Hong Wang. Iptakalim, a novel ATP-sensitive potassium channel opener, inhibits pulmonary arterial smooth muscle cell proliferation by downregulation of PKC-α[J]. The Journal of Biomedical Research, 2011, 25(6): 392-401. DOI: 10.1016/S1674-8301(11)60052-3 |
[10] | Hao Zhang, Hongyan Dong, Bo Jiang, Zheng Wang, Rui Chen, Zhifeng Zhang, Zhongming Zhang. Hypoxic response elements and Tet-On advanced double-controlled systems regulate hVEGF165 and angiopoietin-1 gene expression in vitro[J]. The Journal of Biomedical Research, 2011, 25(3): 204-212. DOI: 10.1016/S1674-8301(11)60027-4 |