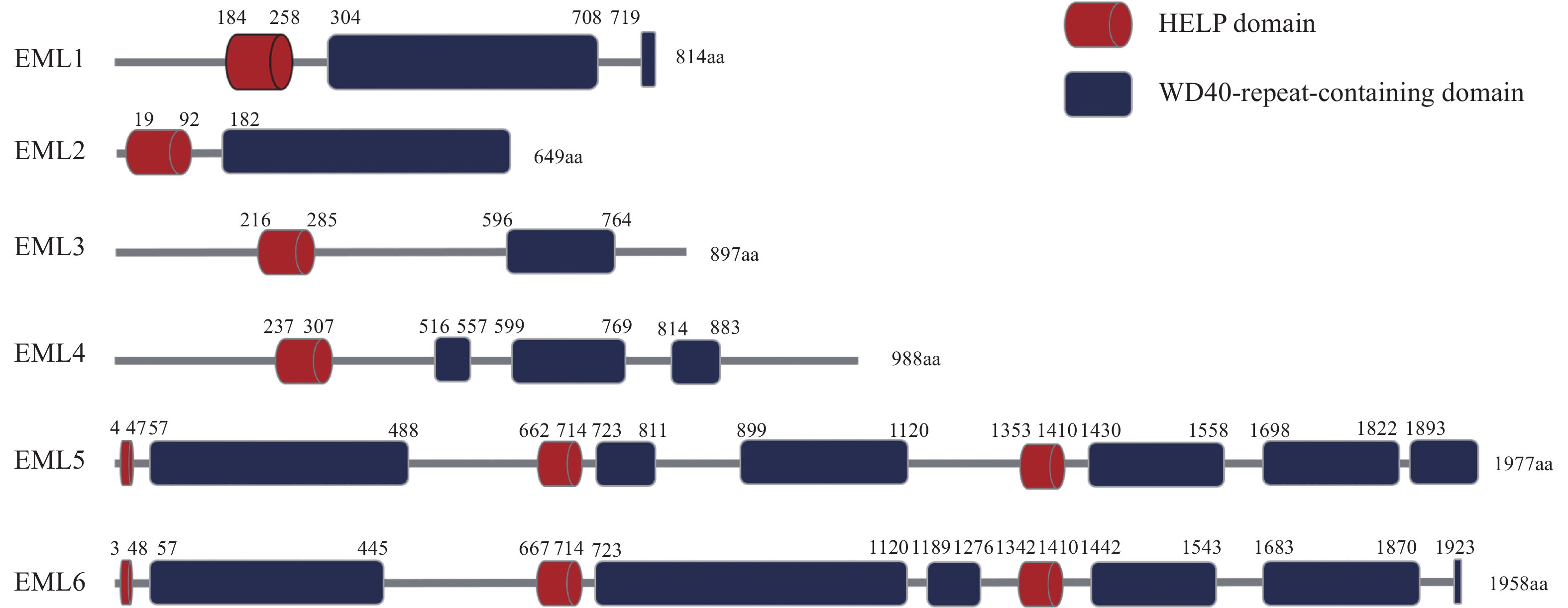
Citation: | Yin Hong, Hou Xuan, Zhang Teng, Shi Lanying, Su You-Qiang. Participation of EML6 in the regulation of oocyte meiotic progression in mice[J]. The Journal of Biomedical Research, 2020, 34(1): 44-53. DOI: 10.7555/JBR.33.20190014 |
To produce an egg competent in fertilization and embryo development, mammalian oocytes must complete meiosis for the recombination and segregation of homologous chromosomes[1]. The precise timing and accurate chromosomal segregation of meiosis are therefore key determinants of oocyte quality. However, for most mammalian species, especially the human, meiosis in oocytes is error-prone, because it frequently causes aneuploidy in the resulting eggs and embryos which is a leading cause for female infertility, miscarriage, and birth defects. As the incidence of aneuploidy rises significantly with the maternal age, there is growing interest in the fundamental mechanisms governing oocyte meiosis and the underlying causes of oocyte aneuploidy[2].
Faithful segregation of chromosomes during oocyte meiotic maturation is heavily dependent upon the formation of a well-organized bipolar spindle apparatus, and the correct interactions between chromosomes and microtubules[3]. Microtubules are made up of hollow cylindrical polymers of α/β-tubulin subunits. These highly dynamic microtubules can switch stochastically between growing and shrinking phases both in vivo and in vitro[4]. The dynamic function of spindles requires the interaction of microtubules with a large number of proteins. These microtubule-associated proteins (MAPs) regulate the stability status of microtubules. For example, some MAPs, such as MAP2[5], Tau[6], and CKAP2[7], promote microtubule growth via stabilizing them; while others, such as stathmin/Op18[8], katanin[9], and XKCM1[10], are microtubule-destabilizing proteins that promote microtubule disassembly. To date, over 200 MAPs are identified to be essential for spindle assembly in somatic cells[11]. However, the composition and function of the meiotic maturation-requiring MAPs in oocytes remain undefined.
Recently, we investigated the potential involvement of mammalian homologs of echinoderm microtubule-associated protein (EMAP), EMLs (EMAP-like), in the control of mouse oocyte meiotic progression. Attention was paid specifically to this group of proteins owing to their uniqueness among the known MAPs. The founding member of the EML family is EMAP, which was first identified in 1993 as the most abundant microtubule-binding protein in sea urchin eggs and embryos[12–13]. The name "EMAP" was then assigned to this protein because of its robust expression in sea urchin, starfish, and sand dollar eggs[12]. The six members of the mammalian EML are highly similar in sequence, and all contain a unique hydrophobic EML protein (HELP) domain required for microtubule binding and several WD40-repeat-containing domains (Fig. 1). Due to lower sequence homology with other known MAPs, such as those in the brain, the mammalian EMLs are considered to be a unique class of MAP. Biochemical studies have indicated that some members, such as EML4, promote microtubule stabilization[14– 16], while others (e.g. EML2) destabilize microtubules[17]. Most EMLs were found to be expressed in the nervous system except EML3, which was also found to be expressed in the liver and the kidney[14–15,18–21]. Despite this yet scant information on the expression and function of EML family members, virtually nothing was known on the expression patterns and the potential roles of EMLs in the ovaries.
Here we reported that EML6 is the only member of the EML family that is preferentially expressed by oocytes in mouse ovaries. EML6 is co-localized with meiotic spindles, and this specialized localization is crucial for the maintenance of spindle integrity and the fidelity of homologous chromosome segregation. To the best of our knowledge, this is the first study to demonstrate the expression and function of the EML family member in meiotic maturation of mammalian oocyte.
Unless otherwise specified, all chemicals and reagents were purchased from Sigma-Aldrich (USA).
Specific pathogen-free (SPF) ICR female mice (9–11 g in weight) were used for all the experiments. These mice were purchased either from Nanjing Medical University Animal Core Facility or Beijing Vital River Laboratory Animal Technology Co., Ltd. All experimental and animal procedures were approved by the Ethical Committee of Laboratory Animals and the Animal Care and Use Committee of Nanjing Medical University, and performed in accordance with the institutional guidelines.
Female mice were primed with 5 U of pregnant mare serum gonadotropin (PMSG) (Ningbo Second Hormone Factory, China) to stimulate the antral follicle development in the ovaries. Ovaries were picked up 46 –48 hours after the initial priming, and placed into a 3 cm dish containing MEM-alpha (Gibco, USA) medium. Large antral follicles were then punctured with a pair of syringes connected with 25 gauge needles to release the mural granulosa cells (MGCs) and cumulus-oocyte complexes (COCs). COCs were collected, and oocytes and cumulus cells were separated by repeatedly pipetting the COCs using a mouth controlled glass pipette with the inner diameter slightly smaller than the oocyte. Similar amount of MGCs, cumulus cells (CCs), and germinal vesicle (GV) stage fully-grown oocytes (FGOs) were then collected. These procedures were carried out in MEM-alpha supplemented with 75 μg/mL penicillin G, 50 μg/mL streptomycin sulfate, 25 μg/mL pyruvate, 3 mg/mL of bovine serum albumin (BSA) and 5 μmol/L milrinone (Calbiochem, Germany), a specific inhibitor of PDE3A to maintain oocyte meiotic arrest.
For oocyte culture, GV-stage oocytes were incubated in MEM (Gibco) supplemented with 75 μg/mL penicillin G, 50 μg/mL streptomycin sulfate, 25 μg/mL pyruvate, 38 μg/mL EDTA and 3 mg/mL BSA. Culture was conducted at 37 °C in an incubator infused with 5% O2, 5% CO2 and 90% N2. Oocytes at GV, Pro-metaphase Ⅰ (Pro-MⅠ), metaphase (MⅠ), anaphase Ⅰ (AⅠ)/telophase Ⅰ (TⅠ), and MⅡ stages were collected at 0, 4, 6–7, 8–10, and 12–14 hours after the culture was set up.
Total RNA was extracted from granulosa cells and oocytes using RNeasy micro kit (Qiagen, Germany), and in vitro transcription and real-time PCR analyses were then carried out using QuantiTect® Reverse Transcription Kit (Qiagen) and QuantiTec® SYBR Green PCR Kits (Qiagen), respectively, on the ABI 7500 Real-time PCR System (Applied Biosystems, USA). The relative fold changes in mRNA levels were calculated via the method of 2−ΔΔCt using Rpl19 as an internal control. The primer sequences for the tested genes are listed in Table 1.
Primers | Sequence (5′→ 3′) |
EML1-F | GCACATCTAAGGATGGAAAGCAA |
EML1-R | CGGTCAAAAAAGCCTATTCCAA |
EML2-F | CCCCGTCACCTGTAAGCAAA |
EML2-R | CCAAATCCCAAACACTCCAAA |
EML3-F | ATTGGTTCCCATGACAACATGA |
EML3-R | TGATAAAACTGGAGTGCCCCATA |
EML4-F | GACGCCAGTGTGACCAAAAC |
EML4-R | GCCCATCCTGCTTTCCTCTT |
EML5-F | CAGATGGCGCTTACCTTGCT |
EML5-R | AAGGGAGCCGACACATTCAC |
EML6-F | AGAAAGACCACCCGTTAGCC |
EML6-R | ATGATGTCGGCACCATCGTT |
Isolated mouse ovaries initially primed with PMSG for 46 hours were fixed for 4 hours in 4% paraformaldehyde, and then embedded in paraffin and sectioned at 5-μm thickness for immunohistochemistry and immunofluorescence. Immunostaining of EML6 was then carried out using rabbit anti-EML6 primary antibody (1:100), and the VECTASTAIN ABC-AP KIT (Rabbit IgG, catalog No. AK-5001, Vector, USA) and VECTOR Red Alkaline Phosphatase (AP) Substrate Kit (catalog no. AK-5100) purchased from Vector Laboratories. Alexa Fluor-488 donkey anti-rabbit IgG was used as the secondary antibody for immunofluorescence.
Oocytes were fixed in 4% paraformaldehyde for 30 minutes at room temperature, and then washed and blocked in PBS containing 10% fetal bovine serum (FBS) and 1% Triton X-100 for 1 hour at 37 °C or at 4 °C overnight. After blocking, oocytes were incubated overnight at 4 °C with EML6 antibody (1:100) and FITC-α-tubulin antibody (1:500, Proteintech, Wuhan, China). After three washes with PBS-1% FBS, oocytes were incubated for 2 hours in Alexa Fluor 594-conjugated secondary antibody solution (1:200), and finally counterstained with DAPI (1:100) for 10 minutes, and mounted on glass slides for imaging using a laser scanning confocal microscope (LSM 700, Carl Zeiss, Germany).
After 8 hours in culture, oocytes at MⅠ stage were transferred to pre-warmed culture medium containing 20 μg/mL nocodazole or 10 μmol/L Taxol, and incubated for 10 or 45 minutes, respectively. Control oocytes were incubated with the same concentration of dimethyl sulfoxide (DMSO) under the same culture conditions. After treatment, the oocytes were washed thoroughly and fixed for immunofluorescence.
Zona pellucida of MⅡ-stage oocytes was removed by exposure to acid Tyrode's solution (pH 2.5) for 30 seconds at 37 °C. The oocytes were put on a glass slide in a small drop of fixative containing 1% PFA and 0.15% Triton X-100, and then air dried. The fixed oocytes were incubated with the Human anti-Centromere (1:500, Antibodies Incorporated, USA) at 4 °C overnight after being blocked with 1% BSA for an hour, followed by incubation with Alexa Fluor594-conjugated donkey anti-Human IgG (1:750, Invitrogen, Shanghai, China). The chromosomes were counterstained with Hoechst 33342 for 15 minutes. The specimens were examined under a LSM 700 laser scanning confocal microscope (Carl Zeiss).
All experiments were repeated at least three times independently, with the data presented as mean ± SEM. Statistical analyses were performed with GraphPad Prism 6.0 (GraphPad Software, USA). Differences between the groups were analyzed using Student's t-test. P<0.05 was considered to be significantly different.
As an initial step to explore the involvement of the mammalian homologue of EMAP in oocyte and ovarian biology, we examined the expression of all six members of the EML family in different compartments within the mouse ovarian follicles. Quantitative real-time RT-PCR analysis revealed that EMLs displayed various patterns of differential expression within the follicles (Fig. 2A). In oocytes, EML4, EML5, and EML6 were expressed at much higher levels than EML1, EML2, and EML3, with EML4 being the most abundantly expressed in oocytes. In follicular somatic cells, i.e., cumulus and mural granulosa cells (CCs and MGCs), all the EMLs were abundantly expressed except that EML6, and EML3 and EML5 were expressed relatively lower than the other three. Interestingly, only EML6 was found to be predominantly expressed by oocytes. The levels of EML6 mRNA in oocytes were 27 and 14 folds higher than those expressed in CCs and MGCs, respectively. Consistent with the preferential expression of the mRNA, immunohistochemistry analysis demonstrated that EML6 protein was also robustly expressed in the oocytes, but barely detected in ovarian somatic cells, including the CCs and MGCs (Fig. 2B).
The predominant expression of EML6 in oocytes implies its important role during oocyte maturation. We therefore investigated the intracellular localization of EML6 during oocyte meiotic progression. As indicated in Fig. 3A, whole mount immunofluorescence revealed that EML6 was ubiquitously distributed in the cytoplasm of GV-stage FGOs. After GV breakdown (GVBD), it was enriched at the spindle region, and co-localized with spindle microtubules at all the meiotic stages that were examined. To ascertain whether the specialized localization of EML6 was dependent on the integrity of the spindle apparatus, MⅠ stage oocytes were treated briefly with spindle de-stabilizer nocodazole and stabilizer Taxol, respectively, and the localization of EML6 was then examined (Fig. 3B). Unlike DMSO treatment which did not affect the size and shape of the MⅠ spindles or EML6 localization, nocodazole treatment disrupted the normal structure of the meiotic spindles, which is coincident with the dissociation of EML6 from the collapsed spindle apparatus. However, Taxol stabilized the MⅠ spindles, and enhanced the co-localization of EML6 with the spindle microtubules.
The specific localization of EML6 on spindles suggests that it may control oocyte meiotic maturation. We tested this possibility by knocking down (KD) the expression of EML6 in GV-stage FGOs and assessing the consequence on oocyte meiotic progression (Fig. 4A). As shown in Fig. 4B, the levels of EML6 mRNA in oocytes were downregulated by 92.5% after receiving the microinjection of EML6-specific small interfering RNAs (siRNAs). GVBD occurred normally, and completed within 1.5–2.0 hours after being released from the milrinone-containing medium in EML6-KD oocytes (Fig. 4C), indicating that EML6 KD did not affect oocyte meiotic resumption. However, the kinetics of the first meiosis, as assessed by emission of the first polar body (PB1), was significantly delayed in EML6-KD oocytes (Fig. 4D).
When the morphology of spindle apparatus and chromosome dynamics were assessed by whole mount IF staining, evident impairment of the alignment of chromosomes and the dimension of spindles was observed in the EML6-KD oocytes both at the MⅠ and MⅡ stages (Fig. 5). Chromosomes were not properly aligned at the spindle equators, and instead were scattering around in EML6-KD oocytes, which resulted in a wider metaphase plate at both the MⅠ- (Fig. 5B) and MⅡ-stages (Fig. 5G). The dimension of meiotic spindles was also distorted in EML6-KD oocytes, with the spindle length and area increased in MⅠ-stage (Fig. 5C and E), while in MⅡ only the width was enlarged (Fig. 5I). Other parameters of the spindles were not changed at both stages (Fig. 5D, H, and J).
The precedent defects in spindle morphology and chromosome alignment observed in EML6-KD oocytes implied that spindle assembly checkpoint (SAC) and the fidelity of homolog separation might also be affected in these oocytes. We therefore tested this possibility by assessing the activation status of SAC in EML6-KD oocytes at MⅠ-stage and the euploidy status of the EML6-KD oocytes that have completed the first meiotic division, respectively. IF staining of BubR1, the SAC component, in oocytes that had been cultured for 7 hours demonstrated that the percentage of chromosomes stained positive for BubR1 was significantly higher in EML6-KD oocytes (Fig. 6A and B), indicating that SAC was constantly active in EML6-KD oocytes when meiosis normally progressed to MⅠ in the control oocytes. Chromosomes spread analysis of the oocytes that have extruded the PB1 revealed that most of the control oocytes had 20 chromosomes, and there were only 5% aneuploid oocytes. In contrast to the control, the EML6-KD oocytes had significant elevated rate of aneuploidy, which increased to nearly 30% (Fig. 6C and D).
Maintenance of the normal structural and functional dynamics of the meiotic spindle is indispensable for meiotic progression and chromosome segregation in mammalian oocytes. Here, we revealed for the first time that the EML family of proteins, a unique class of MAPs, are differentially expressed in the ovarian follicles of mice, with EML1-3 highly expressed by granulosa cells whereas EML4-6 robustly expressed by oocytes. EML6 is the only member that is both abundantly and preferentially expressed in oocytes. Specifically localized to meiotic spindles, it is crucial for the maintenance of spindle dimension and fidelity of homologous chromosome segregation. EML6 is therefore most likely to be a new player in the control of oocyte meiotic division.
We demonstrated here that, at least at mRNA levels, the six members of the EML family were differentially expressed in the follicles of mouse ovaries. EML1-3 were abundantly and preferentially expressed by follicular somatic cells, i.e., cumulus and mural granulosa cells, while EML6 was the only member that was highly and preferentially expressed by oocytes. EML 4 and 5 were abundantly expressed by both granulosa cells and oocytes, with relatively higher levels in oocytes. The compartmentalization of the EML family members in the ovary implies that they may play unique roles in the control of oocyte and granulosa cell development. These data are therefore very informative for exploring the function of EMLs in mammalian ovaries.
Given the robustness and preferential pattern of expression of EML6 in oocytes, we further explored its localization and potential function in oocytes. We found that EML6 was co-localized with spindle microtubules at various stages of meiotic progression after the oocyte resumes meiosis. This is consistent with the spindle localization of most of EML family members during mitosis in somatic cells[16,22]. This meiotic spindle-specific localization was lost when the nucleation of microtubules were disrupted when the oocyte is treated with nocodazole, the microtubule destabilizer; whereas the localization of EML6 on spindles was enhanced by treatment with Taxol, the microtubule stabilizing reagent. These data further buttress the association of EML6 with the microtubules during oocyte meiotic progression, and strongly suggest the participation of EML6 in the control of oocyte meiotic maturation. Indeed, we found that the knockdown of EML6 delayed the progression of meiosis toward metaphase Ⅱ, as indicated by the slower kinetics of extrusion of PB1, although GVB was not affected. This suggests that EML6 is essential for the orderly progression and completion of the first meiosis, but dispensable for the re-initiation of meiosis Ⅰ.
Spindle size and chromosome alignment at spindle equators were also found to be severely impaired in EML6-KD oocytes at both the MⅠ- and MⅡ-stages. This suggests that EML6 is crucial for the assembly and/or maintenance of normal spindles, and the congression of chromosomes during the oocyte meiotic maturation. In accord with the phenotype of chromosome misalignment at MⅠ stages, the rate of BubR1-positive chromosomes within each oocyte was significantly upregulated in EML6-KD oocytes, thus indicating the constant activation of SAC. Therefore, the delay in PB1 extrusion after EML6 knock-down could be explained nicely by chromosome misalignment and SAC activation at MⅠ-stage. Defective segregation of the homologous chromosomes at the end of the first meiosis is frequently reported to lead to chromosome misalignment in the resulting MⅡ-stage oocytes[23–24]. Indeed, we found here that, associated with the defective chromosome alignment and spindle configuration in EML6-KD MⅡ-stage oocytes, the rate of aneuploidy was also increased significantly. This observation suggests that EML6 is also essential for the fidelity of homolog segregation during the final stage of oocyte meiotic maturation. The similar role in chromosome alignment and spindle morphology has also been discovered for EML1, EML3, and EML4 during mitosis in somatic cells[16,22,25], indicating that the members of mammalian EML family play a conserved role in the control of both mitosis and meiosis.
Taken together, although the founding member of EMLs was originally identified from sea urchin eggs and embryos, the participation of mammalian EMLs in the control of oocyte maturation has not been explored previously. It is anticipated that the results presented here will inspire more interest in understanding the roles and related mechanisms of EMLs in the control of oocyte development and maturation.
This study was supported by National Key Research and Development Program (Grant No. 2018YFC1003803) of China; National Natural Science Foundation of China (Grant No. 31871507) to You-Qiang Su. We thank all the members of the Su's laboratory for providing necessary help and assistance in this study.
[1] |
Conti M, Franciosi F. Acquisition of oocyte competence to develop as an embryo: integrated nuclear and cytoplasmic events[J]. Hum Reprod Update, 2018, 24(3): 245–266. doi: 10.1093/humupd/dmx040
|
[2] |
Jones KT, Lane SIR. Molecular causes of aneuploidy in mammalian eggs[J]. Development, 2013, 140(18): 3719–3730. doi: 10.1242/dev.090589
|
[3] |
Baumann C, Wang XT, Yang LH, et al. Error-prone meiotic division and subfertility in mice with oocyte-conditional knockdown of pericentrin[J]. J Cell Sci, 2017, 130(7): 1251–1262. doi: 10.1242/jcs.196188
|
[4] |
Heald R, Nogales E. Microtubule dynamics[J]. J Cell Sci, 2002, 115: 3–4.
|
[5] |
Kalcheva N, Rockwood JM, Kress Y, et al. Molecular and functional characteristics of MAP-2a: ability of MAP-2a versus MAP-2b to induce stable microtubules in COS cells[J]. Cell Motil Cytoskeleton, 1998, 40(3): 272–285. doi: 10.1002/(ISSN)1097-0169
|
[6] |
Stern JL, Lessard DV, Ali R, et al. Single-molecule imaging of Tau dynamics on the microtubule surface[J]. Methods Cell Biol, 2017, 141: 135–154. doi: 10.1016/bs.mcb.2017.06.016
|
[7] |
Jin Y, Murakumo Y, Ueno K, et al. Identification of a mouse cytoskeleton-associated protein, CKAP2, with microtubule-stabilizing properties[J]. Cancer Sci, 2004, 95(10): 815–821. doi: 10.1111/cas.2004.95.issue-10
|
[8] |
Belmont LD, Mitchison TJ. Identification of a protein that interacts with tubulin dimers and increases the catastrophe rate of microtubules[J]. Cell, 1996, 84(4): 623–631. doi: 10.1016/S0092-8674(00)81037-5
|
[9] |
Joly N, Martino L, Gigant E, et al. Microtubule-severing activity of the AAA+ ATPase Katanin is essential for female meiotic spindle assembly[J]. Development, 2016, 143(19): 3604–3614. doi: 10.1242/dev.140830
|
[10] |
Kline-Smith SL, Walczak CE. The microtubule-destabilizing kinesin XKCM1 regulates microtubule dynamic instability in cells[J]. Mol Biol Cell, 2002, 13(8): 2718–2731. doi: 10.1091/mbc.e01-12-0143
|
[11] |
Petry S. Mechanisms of mitotic spindle assembly[J]. Annu Rev Biochem, 2016, 85: 659–683. doi: 10.1146/annurev-biochem-060815-014528
|
[12] |
Suprenant KA, Dean K, McKee J, et al. EMAP, an echinoderm microtubule-associated protein found in microtubule-ribosome complexes[J]. J Cell Sci, 1993, 104(2): 445–550.
|
[13] |
Hamill DR, Howell B, Cassimeris L, et al. Purification of a WD repeat protein, EMAP, that promotes microtubule dynamics through an inhibition of rescue[J]. J Biol Chem, 1998, 273(15): 9285–9291. doi: 10.1074/jbc.273.15.9285
|
[14] |
Houtman SH, Rutteman M, De Zeeuw CI, et al. Echinoderm microtubule-associated protein like protein 4, a member of the echinoderm microtubule-associated protein family, stabilizes microtubules[J]. Neuroscience, 2007, 144(4): 1373–1382. doi: 10.1016/j.neuroscience.2006.11.015
|
[15] |
Pollmann M, Parwaresch R, Adam-Klages S, et al. Human EML4, a novel member of the EMAP family, is essential for microtubule formation[J]. Exp Cell Res, 2006, 312(17): 3241–3251. doi: 10.1016/j.yexcr.2006.06.035
|
[16] |
Chen D, Ito S, Yuan H, et al. EML4 promotes the loading of NUDC to the spindle for mitotic progression[J]. Cell Cycle, 2015, 14(10): 1529–1539. doi: 10.1080/15384101.2015.1026514
|
[17] |
Eichenmuller B, Everley P, Palange D, et al. The human EMAP-like protein-70(ELP70) is a microtubule destabilizer that localizes to the mitotic apparatus[J]. J Biol Chem, 2002, 277(2): 1301–1309. doi: 10.1074/jbc.M106628200
|
[18] |
Kielar M, Tuy FP, Bizzotto S, et al. Mutations in Eml1 lead to ectopic progenitors and neuronal heterotopia in mouse and human[J]. Nat Neurosci, 2014, 17(7): 923–933. doi: 10.1038/nn.3729
|
[19] |
O'Connor V, Houtman SH, De Zeeuw CI, et al. Eml5, a novel WD40 domain protein expressed in rat brain[J]. Gene, 2004, 336(1): 127–137. doi: 10.1016/j.gene.2004.04.012
|
[20] |
Huttlin EL, Jedrychowski MP, Elias JE, et al. A tissue-specific atlas of mouse protein phosphorylation and expression[J]. Cell, 2010, 143(7): 1174–1189. doi: 10.1016/j.cell.2010.12.001
|
[21] |
Villen J, Beausoleil SA, Gerber SA, et al. Large-scale phosphorylation analysis of mouse liver[J]. Proc Natl Acad Sci USA, 2007, 104(5): 1488–1493. doi: 10.1073/pnas.0609836104
|
[22] |
Tegha-Dunghu J, Neumann B, Reber S, et al. EML3 is a nuclear microtubule-binding protein required for the correct alignment of chromosomes in metaphase[J]. J Cell Sci, 2008, 121(Pt 10): 1718–1726.
|
[23] |
Lamb NE, Sherman SL, Hassold TJ. Effect of meiotic recombination on the production of aneuploid gametes in humans[J]. Cytogenet Genome Res, 2005, 111(3-4): 250–255. doi: 10.1159/000086896
|
[24] |
Cao GY, Li MZ, Wang H, et al. Interference with the C-terminal structure of MARF1 causes defective oocyte meiotic division and female infertility in mice[J]. J Biomed Res, 2018, 32(1): 58–67.
|
[25] |
Bizzotto S, Uzquiano A, Dingli F, et al. Eml1 loss impairs apical progenitor spindle length and soma shape in the developing cerebral cortex[J]. Sci Rep, 2017, 7(1): 17308. doi: 10.1038/s41598-017-15253-4
|
[1] | Shiya Xie, Yanjie Yang, Zhen Jin, Xiaocong Liu, Shuping Zhang, Ning Su, Jiaqi Liu, Congrong Li, Dong Zhang, Leilei Gao, Zhixia Yang. Mouse KL2 is a unique MTSE involved in chromosome-based spindle organization and regulated by multiple kinases during female meiosis[J]. The Journal of Biomedical Research, 2024, 38(5): 485-499. DOI: 10.7555/JBR.37.20230290 |
[2] | Xia Wang, Shuai Zhou, Haojie Yin, Jian Han, Yue Hu, Siqi Wang, Congjing Wang, Jie Huang, Junqiang Zhang, Xiufeng Ling, Ran Huo. The role of SRPK1-mediated phosphorylation of SR proteins in the chromatin configuration transition of mouse germinal vesicle oocytes[J]. The Journal of Biomedical Research. DOI: 10.7555/JBR.38.20240054 |
[3] | Cong-Rong Li, Ruo-Lei Wang, Shi-Ya Xie, Yan-Ru Li, Lei-Lei Gao, Zhi-Xia Yang, Dong Zhang. Fidgetin knockdown and knockout influences female reproduction distinctly in mice[J]. The Journal of Biomedical Research, 2022, 36(4): 269-279. DOI: 10.7555/JBR.36.20220086 |
[4] | He Xi, Xie Wenxiu, Li Huiling, Cui Yiqiang, Wang Ya, Guo Xuejiang, Sha Jiahao. The testis-specifically expressed gene Trim69 is not essential for fertility in mice[J]. The Journal of Biomedical Research, 2021, 35(1): 47-60. DOI: 10.7555/JBR.34.20200069 |
[5] | Huang Lei, Lu Qun, Du Jiangbo, Lv Hong, Tao Shiyao, Chen Shiyao, Li Xiuzhu, Han Xiumei, Zhou Kun, Xu Bo, Liu Xiaoyu, Ma Hongxia, Xia Yankai, Jin Guangfu, Shen Hongbing, Ling Xiufeng, Hu Zhibin, Tan Jichun, Diao Feiyang. Cumulative live birth rates of in vitro fertilization/intracytoplasmic sperm injection after multiple complete cycles in China[J]. The Journal of Biomedical Research, 2020, 34(5): 361-368. DOI: 10.7555/JBR.34.20200035 |
[6] | Wu Fang, Liu Feng, Guan Yichun, Du Jiangbo, Tan Jichun, Lv Hong, Lu Qun, Tao Shiyao, Huang Lei, Zhou Kun, Xia Yankai, Wang Xinru, Shen Hongbing, Ling Xiufeng, Diao Feiyang, Hu Zhibin, Jin Guangfu. A nomogram predicting clinical pregnancy in the first fresh embryo transfer for women undergoing in vitro fertilization and intracytoplasmic sperm injection (IVF/ICSI) treatments[J]. The Journal of Biomedical Research, 2019, 33(6): 422-429. DOI: 10.7555/JBR.33.20190065 |
[7] | Kaibo Lin, Shikun Zhang, Jieli Chen, Ding Yang, Mengyi Zhu, Eugene Yujun Xu. Generation and functional characterization of a conditional Pumilio2 null allele[J]. The Journal of Biomedical Research, 2018, 32(6): 434-441. DOI: 10.7555/JBR.32.20170117 |
[8] | Guangyi Cao, Mingzhe Li, Hao Wang, Lanying Shi, You-Qiang Su. Interference with the C-terminal structure of MARF1 causes defective oocyte meiotic division and female infertility in mice[J]. The Journal of Biomedical Research, 2018, 32(1): 58-67. DOI: 10.7555/JBR.32.20170108 |
[9] | Young-Joo Yi, S. Kamala-Kannan, Jeong-Muk Lim, Byung-Taek Oh, Sang-Myeong Lee. Effects of difructose dianhydride (DFA)-IV on in vitro fertilization in pigs[J]. The Journal of Biomedical Research, 2017, 31(5): 453-461. DOI: 10.7555/JBR.31.20160115 |
[10] | Wolfgang J. Schneider. Lipid transport to avian oocytes and to the developing embryo[J]. The Journal of Biomedical Research, 2016, 30(3): 174-180. DOI: 10.7555/JBR.30.20150048 |
Primers | Sequence (5′→ 3′) |
EML1-F | GCACATCTAAGGATGGAAAGCAA |
EML1-R | CGGTCAAAAAAGCCTATTCCAA |
EML2-F | CCCCGTCACCTGTAAGCAAA |
EML2-R | CCAAATCCCAAACACTCCAAA |
EML3-F | ATTGGTTCCCATGACAACATGA |
EML3-R | TGATAAAACTGGAGTGCCCCATA |
EML4-F | GACGCCAGTGTGACCAAAAC |
EML4-R | GCCCATCCTGCTTTCCTCTT |
EML5-F | CAGATGGCGCTTACCTTGCT |
EML5-R | AAGGGAGCCGACACATTCAC |
EML6-F | AGAAAGACCACCCGTTAGCC |
EML6-R | ATGATGTCGGCACCATCGTT |