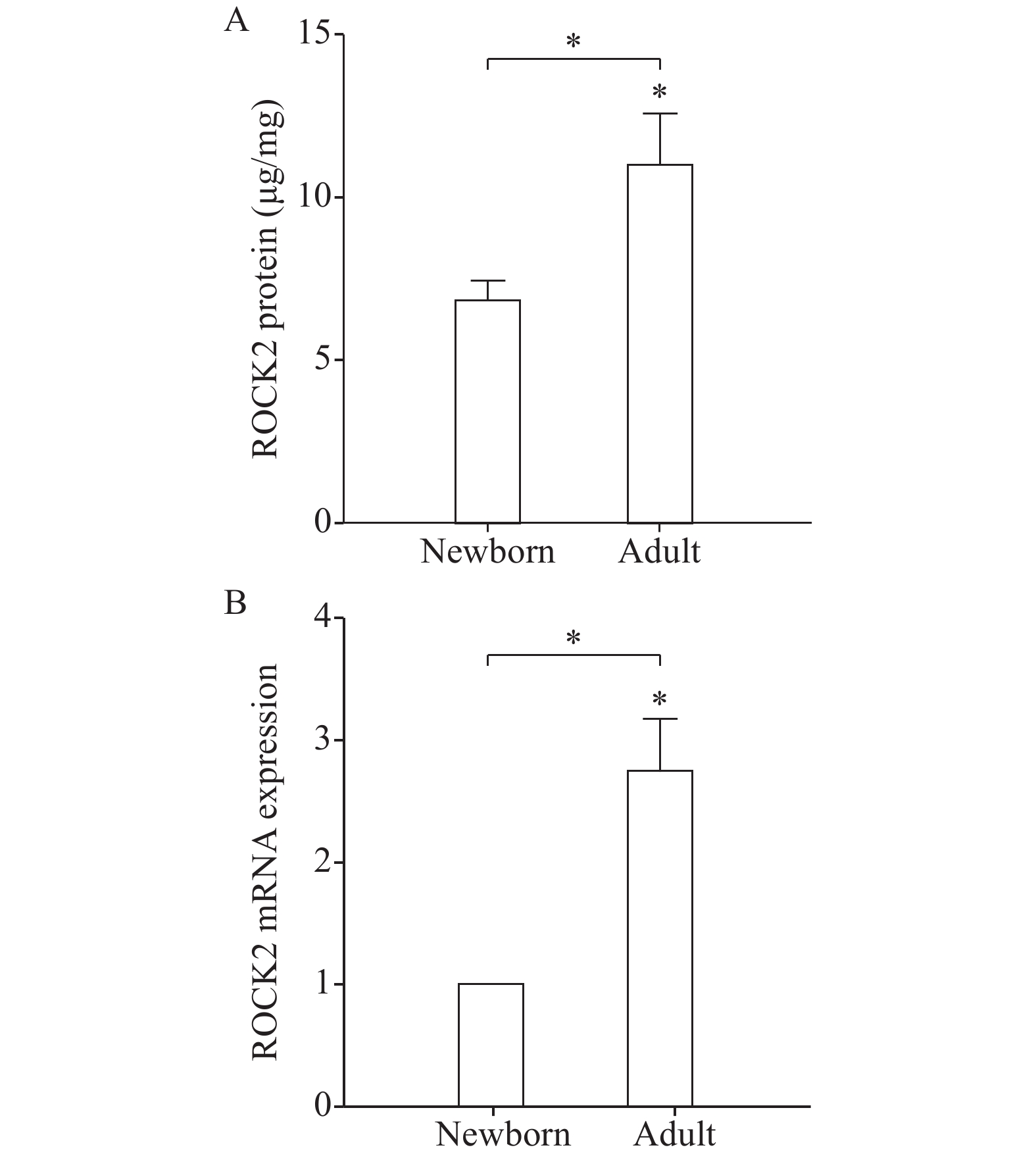
Citation: | Al-Shboul Othman, Alfaqih Mahmoud, Mustafa Ayman, Al-Dwairi Ahmed, Alqudah Mohammad, Haifawi Saja, Alebbini Mohanad, Al-Zaareer Mohammad, Alahmar Khobaib. Developmental changes in contraction of gastric smooth muscle cells in rats correlate with their differences in RhoA/ROCK pathway[J]. The Journal of Biomedical Research, 2019, 33(5): 289-296. DOI: 10.7555/JBR.33.20180086 |
During development from newborn to adult, the gastrointestinal (GI) tract gradually establishes its mature structure and motor function[1−2]. Previous reports have strongly demonstrated delayed emptying and abnormal motility patterns in the stomach of the newborn compared with that of the adult[3−5]. For example, Sobchak et al suggested that gastropyloric motor activity in newborn rats is characterized by a lower pyloric sphincter tone and reduced gastric muscle contraction compared with adult rats[6]. Their findings imply that decreased gastric motor activity, and not increased pyloric sphincter tone, accounts for the reduced gastric content emptying early in life.
Emptying of gastric contents is dependent on a well-coordinated stomach motor function involving gastric smooth muscle contraction and pyloric sphincter relaxation[7]. Improper gastric emptying and motility patterns are common in newborn infants[8−11]. The factors underlying these developmental changes in gastric motility remain unsettled but may involve the neural, hormonal, or myogenic factors that control gastric motility. Researchers have reported that stomach smooth muscle from newborn animals differs from that of the adult with respect to maximal active force development[12−16], sensitivity to agonist stimulation[15], involvement/mobilization of extracellular and intracellular calcium stores[8,12−13,17], and the characteristics of cell membrane calcium channels[12].
Age-dependent changes in agonist-induced force were reported in gastric muscle strips from cats, guinea pigs, and rabbits[12−13,16,18]. These changes were believed to be related to a reduced intracellular Ca2+ mobilization following agonist stimulation in newborn compared with adult gastric muscle[18].
Physiologically, smooth muscle cell is considered the final effector responsible for producing fine and delicate GI tract movements. Phosphorylation of serine 19 on the 20 kDa regulatory light-chain of myosin Ⅱ (MLC20) is an essential and mandatory step in smooth muscle contraction[19−20]. Contraction of smooth muscle is regulated by both Ca2+-dependent and Ca2+-independent (Ca2+ sensitization) mechanisms[21]. An increase in intracellular Ca2+ levels leads to myosin light chain kinase (MLCK) activation, resulting in an increase in MLC20 phosphorylation and thus smooth muscle contraction[22]. More importantly, MLC20 phosphorylation can also be increased through inhibition of MLC phosphatase, which enhances smooth muscle force generation without a change in intracellular Ca2+ level[23]. Rho-associated protein kinase (ROCK), a serine/threonine kinase and an important downstream effector of the small G protein RhoA, has been found to be an important factor in Ca2+ sensitization and developing smooth muscle tone[22−23]. Activation of the RhoA/ROCK pathway maintains the level of MLC20 phosphorylation, the essential step in smooth muscle contraction, via the inhibition of MLC phosphatase activity by phosphorylation of the MLC phosphatase target subunit (MYPT1) at Thr696 by ROCK[24].
Recent study reported that there was increased expression of ROCK2 and MLCK and enhanced phosphorylation of MYPT1 in adult smooth muscle cells of fundus and pyloric sphincter compared to newborn. In addition, expression of the neuronal nitric oxide synthase and phosphorylated vasodilator-stimulated phosphoprotein (important for smooth muscle relaxation) was also elevated in newborn pyloric sphincter smooth muscle cell extracts compared with those of adult animals. Moreover, the potential for force generation and smooth muscle shortening was significantly lower in newborn than adult gastric and pyloric tissues[6].
As understanding changes in gastric motility with age is important clinically in terms of the pathophysiology of disease, we aimed in this study to evaluate the relationship between the catalytic activities and expression of the small monomeric G protein RhoA and ROCK and contraction of stomach smooth muscle cells in early newborn vs. adult rat. Specifically, we tested the hypothesis that the reduced gastric smooth muscle cell contraction in neonatal rats can be accounted for reduced levels and decreased activity of the RhoA/ROCK pathway.
All chemicals, other than those whose suppliers are specifically mentioned above, were obtained from Sigma (St. Louis, MO, USA). The stock solution of Y-27632 was prepared in dimethylsulfoxide.
All experimental protocols were approved by the Animal Care and Use Committee at Jordan University of Science and Technology and all procedures were conducted in accordance with the guidelines set by this committee. Male Sprague-Dawley rats were studied at 1 week (newborn) vs. 12 weeks (adult) of age. Rats were provided by the animal house of the Jordan University of Science and Technology. The rats were housed under standardized conditions (temperature 20–22 °C, humidity 50%–60% and 12 hours light/dark cycle) and allowed free access to food and tap water throughout the experiments. Newborn rats were sacrificed by cervical dislocation while adult rats were euthanized by inhalation of CO2 for 5 minutes followed by an incision through the diaphragm with a scalpel blade for further confirmation of euthanasia. Following euthanasia, the stomach was immediately excised.
GSMCs were isolated from the muscle layer of the rat's stomach by sequential enzymatic digestion, filtration and centrifugation as described previously[25−26]. In brief, strips of muscle from all regions of the stomach were cleaned free of mucosa, dissected, and incubated at 31 °C for 30 minutes in HEPES buffer with the following composition: 120 mmol/L NaCl, 4 mmol/L KCl, 2.0 mmol/L CaCl2, 2.6 mmol/L KH2PO4, 0.6 mmol/L MgCl2, 25 mmol/L HEPES, 14 mmol/L glucose, 2.1% Eagle 's essential amino acid mixture, 0.1% collagenase and 0.01% soybean trypsin inhibitor (pH was adjusted to 7.4). Gassing of the tissue with 100% oxygen was continuously maintained throughout the isolation procedure. Following two washes of the partially digested strips with 50 mL of enzyme-free medium, the muscle cells were allowed to disperse spontaneously for 30 minutes. Filtration through 500-μm Nitex mesh was used to harvest the cells which were then centrifuged twice at 350 g for 10 minutes to eliminate broken cells and organelles. This cell isolation procedure consistently yielded spindle-shaped and viable GSMCs that exhibited notable contraction in response to contractile stimuli. All the experiments were performed within 2–3 hours of cell dispersion.
The membranes of the collected GSMCs were broken down by repeated freeze-thaw cycles. After centrifugation of the lysates (20 000 g, 10 minutes, 4 °C), the protein concentrations of the supernatant were determined using a DC Protein Assay kit from Bio-Rad Laboratories (Hercules, CA, USA). Samples of equal amounts of proteins were quantitated for ROCK2 protein using enzyme-linked immunosorbent assays (ELISA) according to the manufacturer 's instructions [ROCK2 ELISA kit (CSBEL020059RA), Cusabio Biotech, Newark, DE, USA].
Real-time PCR was performed on cDNA samples synthesized from total RNA isolated from stomach muscle cells with Real-time PCR Detection System (Insta Q96-LA1012) and intercalating dye, SYBR green. PCR conditions were optimized on the gradient thermal cycler on BioRad T100 PCR Thermal Cycler. For each cDNA sample, real-time PCR was conducted in a 20 μL reaction volume containing SYBR green PCR Master mix (KAPA-Biosystems, Boston, MA, USA). The following time and temperature profile was used for real-time PCR reactions: 95 °C for 3 minutes; 40 cycles of a series consisting of 3 seconds at 95 °C, 20 seconds at 60 °C, and 30 seconds at 72 °C. The optimal annealing temperatures were determined empirically for each primer set. The sequences of specific primers for RhoA F: 5′-GGCAGAGATATGGCAAACAGG-3′, R: 5′-TCCGTCTTTGGTCTTTGCTGA-3′ and for ROCK2 are F: 5′-CCCGATCATCCCCTAGAACC-3′, R: 5′-TTGGAGCAAGCTGTCGACTG-3 ′. Real-time PCR reactions were performed in triplicate. Each primer set generated only one PCR product, and the identity and integrity of these products were confirmed by electrophoresis on 1.5% agarose gel containing 0.1 μg/mL ethidium bromide and sequencing of the individual bands. The fluorescent threshold value was calculated by Real-time PCR Detection System software. The absence of peaks in water controls suggested a lack of primer-dimer formation. GAPDH was selected as reference gene. Cycle threshold (Ct) values were obtained and the relative fold change in gene expression was calculated as 2−ΔΔCt.
ROCK activity was analyzed by enzyme immunoassay with Cell Biolabs ' 96-well ROCK activity assay kit (STA-416, Cell Biolabs Inc., San Diego, CA, USA) according to the manufacturer's protocol. Briefly, GSMCs were broken down by repeated freeze-thaw cycles. GSMCs lysates containing equal proteins were then incubated in a 96-well plate precoated with MYPT1, a ROCK substrate, in the presence of kinase reaction buffer containing ATP for 60 minutes at 30 °C. After the plates were washed with the washing buffer provided in the kit, the wells were incubated with anti-phospho-MYPT1 (Thr696) antibody at room temperature for 1 hour. The wells were then extensively washed, and a horseradish peroxidase (HRP)-conjugated secondary antibody was added for another hour at room temperature. Substrate solution was then added to the wells and quantification performed using a BioTek microplate reader at 450 nm as the primary wavelength.
Levels of activated and total RhoA were determined using the RhoA G-LISATM Activation Assay kit (BK124) and the Total RhoA ELISA kit (BK150) (Cytoskeleton Inc., Denver, CO, USA), respectively, according to the manufacturer's instructions. Briefly, GSMCs were lysed using the provided cell lysis buffer and the protease inhibitor cocktail in the kits. Lysates were quantified using the Bio-Rad DC Protein Assay kit by measuring the absorbance at 750 nm on the BioTek microplate reader. GSMCs lysates containing equal proteins were loaded onto the G-LISA (activated RhoA) or ELISA (total RhoA) plate for protein analysis. The results were obtained as absorbance values at 490 nm. For total RhoA, the results were converted to total protein levels using a standard curve obtained with each test.
Contraction in freshly dispersed GSMCs was determined by scanning micrometry[26]. Aliquots (0.4 mL) of cells containing approximately 1 × 104 cells/mL were prepared and categorized into aliquots of cells from either newborn or adult rats. Cells were stimulated with acetylcholine (ACh, 0.1 μmol/L) for 10 minutes at room temperature in the presence or absence of the ROCK inhibitor Y-27632 (1 μmol/L) (Santa Cruz Biotechnology, Dallas, TX), and the reaction was terminated with the addition of 1% acrolein at a final concentration of 0.1%. Acrolein kills and fixes cells without affecting cell length. The cells were viewed using a 10 × or 20 × objective on an inverted Nikon TMS-f microscope (Nikon Corp., Tokyo, Japan), and cell images were acquired using a Canon digital camera (DS126291, Canon Inc., Tokyo, Japan) and ImageJ acquisition software (v1.45, National Institutes of Health, Bethesda, MD, USA). The resting cell length was determined in control experiments in which muscle cells were not treated with ACh. The mean length of 50 muscle cells from each group was measured by ImageJ software. The contractile response to ACh was defined as the decrease in the mean length of 50 cells and expressed as the percentage of change in length relative to the mean resting length.
Results are expressed as the mean±standard error of the mean (SEM). Statistical analysis of all experiments was performed using Prism 5.0 software (GraphPad Software, San Diego, CA, USA). Comparisons between groups were analyzed by Student's t-test. A P<0.05 was considered statistically significant.
To investigate whether ROCK pathway is developmentally regulated, we compared the protein levels of ROCK2, the predominant smooth muscle isoforms[27], in GSMCs isolated from adult and newborn rats by ELISA. The expression of ROCK2 proteins were elevated in adult gastric muscle cells compared to newborn ones (P<0.05, Fig. 1A). Moreover, real-time PCR results showed also increased ROCK2 mRNA expression in adult GSMCs compared to newborn cells (P<0.05, Fig. 1B).
Treatment of freshly dispersed adult and newborn GSMCs with 0.1 μmol/L ACh, a Gαq/13-coupled receptor agonist, for 10 minutes significantly increased ROCK activity above the basal level. Importantly, ACh-induced ROCK activity was elevated in adult versus newborn GSMCs (P<0.05) (Fig. 2). Basal ROCK activity was similar in both newborn and adult groups of cells (data not shown).
Next, we tested the possibility that differences in ROCK activity might be due to an effect on other upstream regulators of the enzyme, such as RhoA. Treatment of freshly dispersed adult and newborn GSMCs with 0.1 μmol/L ACh for 10 minutes significantly augmented RhoA activation above the basal level. Most importantly, ACh-induced RhoA activation was elevated in adult GSMCs than in newborn ones (P<0.05, Fig. 3A). Basal RhoA activity was similar in the two groups of isolated from male and female rats (data not shown). Consistent with its activation profile, levels of total RhoA protein (active and inactive forms) and mRNA were higher in adult GSMCs than in newborn cells (P<0.05, Fig. 3B and C).
Freshly dispersed GSMCs from adult and newborn rats were treated with 0.1 μmol/L ACh, and the decrease in muscle cell length was measured by scanning micrometry. The lengths of resting muscle cells from male and female rats were similar (data not shown). ACh caused both newborn and adult muscle cells to contract, but adult muscle cells showed a significantly more contraction than newborn cells (P<0.05, Fig. 4). Preincubation of GSMCs with the ROCK inhibitor Y-27632 (1 μmol/L), significantly reduced ACh-induced contraction in the muscles cells of both groups (P<0.01) and most importantly, the ROCK inhibitor abolished the reported differences in contractions (Fig. 4).
Gastrointestinal smooth muscle has been shown to have a reduced ability to generate contraction during the newborn period, and research has reported that newborn gastric muscle from various species generate less contraction than the adult gastric muscle[6,12,18]. Moreover, gastric smooth muscle from newborn animals has been demonstrated to undergo a period of postnatal maturation with respect to agonist sensitivity[15] and Ca2+ signaling[14,16], and that agonist-induced contraction may follow distinct intracellular pathways in the adult and newborn. Functionally, this maturational muscle behavior fits well the period of transition in newborn's diet from liquid breast milk to a diet that is a mixture of solids and liquids which increases the burden on the gastric muscle with age. Indeed, a large number of clinical problems in the newborn relating to upper GI motor function were reported. For example, gastroesophageal reflux and vomiting are common during the first year of life[27].
As the gastric motor activity reflects the integrated maturation of the wall muscle, enteric and extrinsic nervous system, interstitial cells of Cajal and hormonal factors[28], we evaluated freshly dispersed SMCs, thus allowing us to comparatively determine their motor activity independently of the remaining external non-muscle factors. Our cell isolation technique has been extensively used for years to yield only dispersed smooth muscle cells indicated by the specific staining with anti-calponin (h1-calponin is specific to differentiated smooth muscle cells) as shown in our previous publications[29−31].
In the present study, we found that contraction (as indicated by cell shortening) is significantly lower in newborn than adult stomach smooth muscle cells. In addition, we documented that the expression and activation of the RhoA/ROCK pathway, an important regulator of smooth muscle tone, is higher in adult than in newborn gastric smooth muscle cells.
Contraction of smooth muscle involves two mechanisms. The first is Ca2+-dependent and manifested by a rise of intracellular Ca2+ which stimulates MLC phosphorylation via MLCK, resulting in muscle contraction[20]. Age-dependent differences in gastric muscle Ca2+ mobilization[18] and MLCK expression[6] were demonstrated. The second mechanism has been shown to be more important than intracellular Ca2+ changes in the stomach muscle cells and is called Ca2+ sensitization[32]. This involves muscle contraction via MLC phosphatase (MLCP) inhibition[33]. More importantly, activation of the RhoA/ROCK pathway inhibits MLCP activity via phosphorylation of the regulatory subunit MYPT1 and induces Ca2+ sensitization[24,34]. Two isoforms of ROCK have been identified in mammalian cells, ROCK1 (or α isoform) and ROCK2 (or β isoform). We examined the expression of ROCK2 isoform as it is functionally more important in the GI tract[34−37]. For measuring ROCK activation, we used ROCK assay which uses MYPT1 Thr696 as a probe for the kinase which can be phosphorylated by ROCK. Our findings of higher ROCK2 expression and activity in adult stomach muscle cells than newborn ones are in consistence with the findings reported by Sobchak et al[6]. Their work and ours suggest that, aside from the reported reduced Ca2+ mobilization early in life, age-dependent changes in Ca2+ sensitization also contribute to less gastric muscle shortening potential in the newborn than adult rat.
We further examined differences in the activation of RhoA, the upstream regulator of the ROCK enzyme. The cytosolic inactive form of RhoA (RhoA.GDP) is bound to a guanine dissociation inhibitor (GDI). RhoA is activated upon GTP binding and thus it interacts with and stimulates the activity of downstream effectors, including ROCK [24]. Parallel to ROCK results, both total RhoA and activated RhoA (GTP-bound) were more in adult stomach smooth muscle cells compared to newborn. A similar trend of increased RhoA expression and activation with age was reported in other non-GI smooth muscle regions[38]. Functionally, smooth muscle plays an important role in stomach wall contraction. Gastric emptying is an age-dependent function and is reported to be, compared with adults, delayed in newborns[3−4,24]. Our reduced muscle contraction in the newborn compared with the adult at the single cell level reinforces this widely accepted stomach behavior. Interestingly, treating muscle cells with Y-27632, a well-known ROCK inhibitor[39], induced stronger inhibition of muscle cell contraction in adult (~67.5%) compared to newborn (~59.8%) cells and greatly abolished ACh-stimulated contraction differences. This strongly proves the contribution of RhoA/ROCK pathway in this age-dependent contractile activity.
In conclusion, our data suggest that stomach muscle cell activity in newborn rats is best characterized by reduced contraction and lower RhoA/ROCK pathway activation compared with adult animals. Whether the maturational changes that occur in our rat stomach smooth muscle cells are relevant to the human condition is not known and has to be tested. Further understanding of age-dependent changes in gastric muscle contraction and RhoA/ROCK and other signaling pathways will facilitate the generation of more effective and age-tailored therapeutic strategies for various GI disturbances.
This work was supported by Jordan University of Science & Technology, Irbid, Jordan (Grant No. 20180087).
[1] |
Anderson RB, Enomoto H, Bornstein JC, et al. The enteric nervous system is not essential for the propulsion of gut contents in fetal mice[J]. Gut, 2004, 53(10): 1546–1547.
|
[2] |
Burns AJ, Roberts RR, Bornstein JC, et al. Development of the enteric nervous system and its role in intestinal motility during fetal and early postnatal stages[J]. Semin Pediatr Surg, 2009, 18(4): 196–205. doi: 10.1053/j.sempedsurg.2009.07.001
|
[3] |
Ramirez A, Wong WW, Shulman RJ. Factors regulating gastric emptying in preterm infants[J]. J Pediatr, 2006, 149(4): 475–479. doi: 10.1016/j.jpeds.2006.05.028
|
[4] |
Riezzo G, Indrio F, Montagna O, et al. Gastric electrical activity and gastric emptying in term and preterm newborns[J]. Neurogastroenterol Motil, 2000, 12(3): 223–229. doi: 10.1046/j.1365-2982.2000.00203.x
|
[5] |
Kasirer MY, Welsh C, Pan JY, et al. Metoclopramide does not increase gastric muscle contractility in newborn rats[J]. Am J Physiol Gastrointest Liver Physiol, 2014, 306(5): G439–G444. doi: 10.1152/ajpgi.00242.2013
|
[6] |
Sobchak C, Fajardo AF, Shifrin Y, et al. Gastric and pyloric sphincter muscle function and the developmental-dependent regulation of gastric content emptying in the rat[J]. Am J Physiol Gastrointest Liver Physiol, 2016, 310(11): G1169–G1175. doi: 10.1152/ajpgi.00046.2016
|
[7] |
Camilleri M, Parkman HP, Shafi MA, et al. Clinical guideline: management of gastroparesis[J]. Am J Gastroenterol, 2013, 108(1): 18–37. doi: 10.1038/ajg.2012.373
|
[8] |
Ittmann PI, Amarnath R, Berseth CL. Maturation of antroduodenal motor activity in preterm and term infants[J]. Dig Dis Sci, 1992, 37(1): 14–19. doi: 10.1007/BF01308336
|
[9] |
Berseth CL, Ittmann PI. Antral and duodenal motor responses to duodenal feeding in preterm and term infants[J]. J Pediatr Gastroenterol Nutr, 1992, 14(2): 182–186. doi: 10.1097/00005176-199202000-00011
|
[10] |
Cavell B. Gastric emptying in preterm infants[J]. Acta Paediatr Scand, 1979, 68(5): 725–730. doi: 10.1111/j.1651-2227.1979.tb18446.x
|
[11] |
Siegel M. Gastric emptying time in premature and compromised infants[J]. J Pediatr Gastroenterol Nutr, 1983, 2 Suppl 1: S136–S140.
|
[12] |
Zitterman J, Ryan JP. Development of gastric antral smooth muscle contractility in newborn rabbits[J]. Am J Physiol, 1990, 258(4): G571–G575.
|
[13] |
Paul DA, Ierardi JA, Parkman HP, et al. Developmental changes in gastric fundus smooth muscle contractility and involvement of extracellular calcium in fetal and adult guinea pigs[J]. Pediatr Res, 1994, 36(5): 642–646. doi: 10.1203/00006450-199411000-00019
|
[14] |
Hyman PE, Martin MG, Tomomasa T, et al. Development of calcium channels in gastric smooth muscle[J]. Pediatr Res, 1989, 25(6): 600–604. doi: 10.1203/00006450-198906000-00010
|
[15] |
Tomomasa T, Yagi H, Kimura S, et al. Developmental changes in agonist-mediated gastric smooth muscle contraction in the rabbit[J]. Pediatr Res, 1989, 26(5): 458–461. doi: 10.1203/00006450-198911000-00019
|
[16] |
Hillemeier AC, Bitar KN, Biancani P. Developmental characteristics of the kitten antrum[J]. Gastroenterology, 1991, 101(2): 339–343. doi: 10.1016/0016-5085(91)90009-A
|
[17] |
Somlyo AP, Somlyo AV. Signal transduction by G-proteins, rho-kinase and protein phosphatase to smooth muscle and non-muscle myosin II[J]. J Physiol, 2000, 522(2): 177–185. doi: 10.1111/tjp.2000.522.issue-2
|
[18] |
Hillemeier AC, Deutsch DE, Bitar KN. Signal transduction pathways associated with contraction during development of the feline gastric antrum[J]. Gastroenterology, 1997, 113(2): 507–513. doi: 10.1053/gast.1997.v113.pm9247470
|
[19] |
Hartshorne DJ, Ito M, Erdödi F. Myosin light chain phosphatase: subunit composition, interactions and regulation[J]. J Muscle Res Cell Motil, 1998, 19(4): 325–341. doi: 10.1023/A:1005385302064
|
[20] |
Somlyo AP, Somlyo AV. Ca2+ sensitivity of smooth muscle and nonmuscle myosin II: modulated by G proteins, kinases, and myosin phosphatase[J]. Physiol Rev, 2003, 83(4): 1325–1358. doi: 10.1152/physrev.00023.2003
|
[21] |
Somlyo AP, Somlyo AV. Signal transduction and regulation in smooth muscle[J]. Nature, 1994, 372(6503): 231–236. doi: 10.1038/372231a0
|
[22] |
de Godoy MAF, Rattan S. Role of rho kinase in the functional and dysfunctional tonic smooth muscles[J]. Trends Pharmacol Sci, 2011, 32(7): 384–393. doi: 10.1016/j.tips.2011.03.005
|
[23] |
Kitazawa T, Gaylinn BD, Denney GH, et al. G-protein-mediated Ca2+ sensitization of smooth muscle contraction through myosin light chain phosphorylation[J]. J Biol Chem, 1991, 266(3): 1708–1715.
|
[24] |
Murthy KS. Signaling for contraction and relaxation in smooth muscle of the gut[J]. Annu Rev Physiol, 2006, 68: 345–374. doi: 10.1146/annurev.physiol.68.040504.094707
|
[25] |
Murthy KS, Makhlouf GM. Interaction of cA-kinase and cG-kinase in mediating relaxation of dispersed smooth muscle cells[J]. Am J Physiol, 1995, 268(1): C171–C180. doi: 10.1152/ajpcell.1995.268.1.C171
|
[26] |
Al-Shboul OA, Al-Dwairi AN, Alqudah MA, et al. Gender differences in the regulation of MLC20 phosphorylation and smooth muscle contraction in rat stomach[J]. Biomed Rep, 2018, 8(3): 283–288.
|
[27] |
Hillemeier AC. Gastroesophageal reflux: diagnostic and therapeutic approaches[J]. Pediatr Clin North Am, 1996, 43(1): 197–212. doi: 10.1016/S0031-3955(05)70402-8
|
[28] |
Sanders KM, Koh SD, Ro S, et al. Regulation of gastrointestinal motility-insights from smooth muscle biology[J]. Nat Rev Gastroenterol Hepatol, 2012, 9(11): 633–645. doi: 10.1038/nrgastro.2012.168
|
[29] |
Al-Shboul O. The role of the RhoA/ROCK pathway in gender-dependent differences in gastric smooth muscle contraction[J]. J Physiol Sci, 2016, 66(1): 85–92. doi: 10.1007/s12576-015-0400-9
|
[30] |
Al-Shboul O, Mustafa A. Effect of oxidative stress on Rho kinase II and smooth muscle contraction in rat stomach[J]. Can J Physiol Pharmacol, 2015, 93(6): 405–411. doi: 10.1139/cjpp-2014-0505
|
[31] |
Al-Shboul OA, Nazzal MS, Mustafa AG, et al. Estrogen relaxes gastric muscle cells via a nitric oxide- and cyclic guanosine monophosphate-dependent mechanism: a sex-associated differential effect[J]. Exp Ther Med, 2018, 16(3): 1685–1692.
|
[32] |
Bhetwal BP, An CL, Fisher SA, et al. Regulation of basal LC20 phosphorylation by MYPT1 and CPI-17 in murine gastric antrum, gastric fundus, and proximal colon smooth muscles[J]. Neurogastroenterol Motil, 2011, 23(10): e425–e436. doi: 10.1111/nmo.2011.23.issue-10
|
[33] |
Kureishi Y, Kobayashi S, Amano M, et al. Rho-associated kinase directly induces smooth muscle contraction through myosin light chain phosphorylation[J]. J Biol Chem, 1997, 272(19): 12257–12260. doi: 10.1074/jbc.272.19.12257
|
[34] |
Berridge MJ. Smooth muscle cell calcium activation mechanisms[J]. J Physiol, 2008, 586(21): 5047–5061. doi: 10.1113/jphysiol.2008.160440
|
[35] |
Dhaese I, Lefebvre RA. Myosin light chain phosphatase activation is involved in the hydrogen sulfide-induced relaxation in mouse gastric fundus[J]. Eur J Pharmacol, 2009, 606(1–3): 180–186. doi: 10.1016/j.ejphar.2009.01.011
|
[36] |
Huang JA, Zhou HP, Mahavadi S, et al. Signaling pathways mediating gastrointestinal smooth muscle contraction and MLC20 phosphorylation by motilin receptors[J]. Am J Physiol Gastrointest Liver Physiol, 2005, 288(1): G23–G31. doi: 10.1152/ajpgi.00305.2004
|
[37] |
Rattan S, Phillips BR, Maxwell IV PJ. RhoA/Rho-kinase: pathophysiologic and therapeutic implications in gastrointestinal smooth muscle tone and relaxation[J]. Gastroenterology, 2010, 138(1): 13–18.e3. doi: 10.1053/j.gastro.2009.11.016
|
[38] |
Bailly K, Ridley AJ, Hall SM, et al. RhoA activation by hypoxia in pulmonary arterial smooth muscle cells is age and site specific[J]. Circ Res, 2004, 94(10): 1383–1391. doi: 10.1161/01.RES.0000128405.83582.2e
|
[39] |
Uehata M, Ishizaki T, Satoh H, et al. Calcium sensitization of smooth muscle mediated by a Rho-associated protein kinase in hypertension[J]. Nature, 1997, 389(6654): 990–994. doi: 10.1038/40187
|