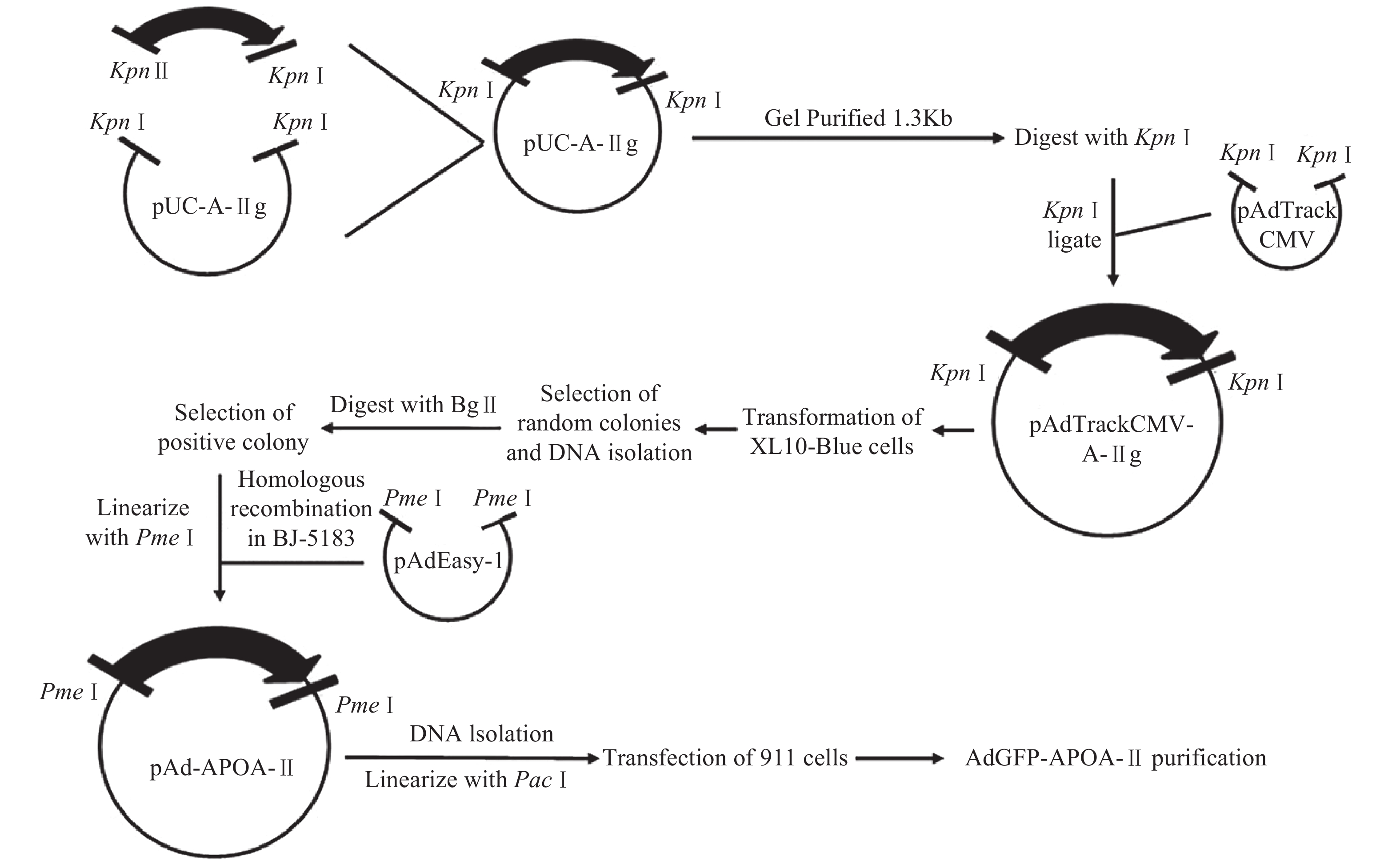
Citation: | Zvintzou Evangelia, Xepapadaki Eva, Kalogeropoulou Christina, Filou Serafoula, Kypreos Kyriakos E.. Pleiotropic effects of apolipoprotein A-Ⅱ on high-density lipoprotein functionality, adipose tissue metabolic activity and plasma glucose homeostasis[J]. The Journal of Biomedical Research, 2020, 34(1): 14-26. DOI: 10.7555/JBR.33.20190048 |
Apolipoprotein A-Ⅱ (APOA-Ⅱ) is the second most abundant apolipoprotein of high-density lipoprotein (HDL)[1], composed of 77 amino acids and synthesized mainly by the liver and to a much lesser extent by the intestine. It has been proposed that APOA-Ⅱ plays a crucial role in HDL particle synthesis, composition and function[2]. In vitro studies indicated that ΑPOA-Ⅱ forms dimers with apolipoprotein E (APOE) thus affecting the ability of APOE to associate with HDL particles[3]. Transgenic mice that overexpress human APOA-Ⅱ had abnormal lipoprotein composition, increased high-density lipoprotein cholesterol (HDL-C) levels and were prone to atherosclerosis[4]. In contrast, studies in human thus far failed to establish a clear role for APOA-Ⅱ in coronary heart disease (CHD). In some studies, APOA-Ⅱ is shown to promote the development of atherosclerosis[5]. However, in a study of 126 subjects with varying degrees of atherosclerosis (calcified and non-calcified), APOA-Ⅱ appeared to positively associate with reverse cholesterol transport and negatively associate with non-calcified atherosclerosis burden[6].
HDL-C has been considered a marker for atheroprotection[7]. The inverse correlation between HDL-C levels and the risk for developing CHD supported by numerous epidemiological studies[8] led to the prevailing view that high HDL is protective against the development of atherosclerosis. However, the failure of investigational drugs such as inhibitors against cholesterol-ester transfer protein (CETP) (torcetrapib[9], dalcetrapib[10] and evacetrapib[11]) to demonstrate clinical efficacy provided for the first time tangible evidence that challenged the classical view on the predictive role of HDL-C levels in atheroprotection. The failure of high-dose niacin, another HDL raising drug, to reduce the risk for cardiovascular events (AIM-HIGH[12] and HPS2-THRIVE[13] clinical trials), also provided additional evidence that simply raising HDL-C in plasma is not an effective strategy for the prevention and treatment of CHD as once believed. These results, along with Mendelian randomization studies failing to demonstrate a causative relationship between HDL-C and cardiovascular diseases, and more recent epidemiological data demonstrating a U-shape correlation between all-cause mortality and HDL-C levels, further supported that excessive increase in HDL-C may be detrimental to human health[14]. More recent findings from mouse studies and clinical trials indicate that HDL functionality, as determined by its lipidome and proteome, is far more important in atheroprotection than HDL-C levels alone[8].
Even though HDL is usually called the "good cholesterol", it is actually more than just a "cholesterol". HDL particles may be of discoidal or spherical shapes with densities in the range of 1.063 to 1.21 g/mL, composed of apolipoproteins, enzymes and lipids. The main protein component of HDL is apolipoprotein A1 (APOA-Ⅰ) which plays a key role in the biogenesis and functions of HDL[15]. In addition, APOE-containing-HDL (APOE-HDL) and APOC-Ⅲ-containing-HDL (APOC-Ⅲ-HDL) particles that are functionally distinct from APOA-Ⅰ-containing-HDL (APOA-Ⅰ-HDL) may also be found[16–18]. The apparent differences in HDL apolipoprotein content, lipidome and functionality between APOE-HDL, APOC-Ⅲ-HDL and APOA-Ⅰ-HDL, identified previously, reinforced our theory that not all HDL particles are equally active and that HDL proteome dictates its lipidome and subsequently its functionality[17]. In support of this theory, another recent study indicated that genetic control of the mouse HDL proteome defines HDL traits, function, and heterogeneity[19].
Thus, HDL proteome appears to influence to a great extent its properties and functions with respect to human physiology[20– 21]. In addition to its role in atherosclerosis, HDL has also been implicated in the regulation of adipose tissue metabolic activation and plasma glucose homeostasis[7]. However, up to this date, the assignment of a clear role of APOA-Ⅱ in HDL functionality remains an unexplored area that may provide significant new mechanistic insights for human health and disease.
To address this question, we investigated the effects of increased APOA-Ⅱ expression on HDL structure and function, adipose tissue metabolic activity, glucose tolerance and insulin sensitivity using adenovirus-mediated gene transfer of human APOA-Ⅱ to C57BL/6 mice. Our combined data from biochemical and molecular analyses indicate that APOA-Ⅱ expression has pleiotropic effects including distinct alterations in HDL particle structure and functionality, improvement of plasma glucose tolerance and select changes in white adipose tissue (WAT) mitochondrial metabolic activation.
C57BL/6 mice aged 20–24 weeks were allowed unrestricted access to food (standard diet) and water under a 12-hour light/dark cycle (7:00 a.m.– 6:59 p.m. light). Sample size was determined based on the desired power of statistical analysis, using an online statistical tool (http://www.stat.ubc.ca/~rollin/stats/ssize/n2.html) as indicated in each experiment. All animal experiments were conducted according to the EU guidelines of the Protocol for the Protection and Welfare of Animals. The work was authorized by the Laboratory Animal Centre committee of The University of Patras Medical School and the Veterinary Authority of the Prefecture of Western Greece.
The genomic DNA sequence containing exons Ⅱ, Ⅲ, Ⅳ of the human APOA-Ⅱ gene was amplified and extracted by PCR (polymerase chain reaction), using the following primers: APOA-Ⅱ-sense (5′-CGGGGTACCGCCCATTTGGCTTCAGTGTCCCCCA-3 ′) and APOA-Ⅱ-antisense (5′-CGGGGTACCCCTCCCTTACTCCCCTACTTTTCC-3′). Both primers carried the KpnI restriction site on their 5′ end. The strategy for the construction of the recombinant attenuated adenovirus expressing human APOA-Ⅱ (AdGFP-APOA-Ⅱ) is summarized in Fig. 1. Recombinant adenovirus was constructed by homologous recombination in BJ-5183 bacterial cells using the Ad-Easy-1 system. Electroporated mixtures were plated on kanamycin plates and the next day small colonies were picked and grown in mini cultures. DNA was isolated and analyzed on a 1% agarose gel. DNA from the selected, as positive, colony was linearized with PacI
Following plaque identification and isolation, adenoviruses were expanded in HEK293 cells and then purified by double CsCl ultracentrifugation, followed by dialysis and titration of the recombinant adenoviruses. The title obtained was approximately (5×1013 pfu/L).
Human astrocytoma cells (SW 1783, ATCC® HTB13TM) were infected with AdGFP or AdGFP-APOA-Ⅱ at a multiplicity of infection of 20. After 48 hours, the culture medium was collected and analyzed.
Mice were injected intravenously through the tail vein with a dose of 2×109 pfu of AdGFP or AdGFP-APOA-Ⅱ. Following four hours of fasting, blood and tissue samples were isolated five days post-infection. To assess the expression of APOA-Ⅱ in the infected mice, total hepatic RNA was analyzed by Northern blotting, and plasma by Western blotting for APOA-Ⅱ mRNA and APOA-Ⅱ protein respectively.
Total cholesterol levels were assessed spectrophotometrically in plasma samples and lipoprotein fractions, using the DiaSys Cholesterol FS kit (ref# 11300, Diagnostic Systems, GmbH, Holzheim, Germany) according to manufacturers' instructions. Triglyceride and phospholipid levels were also assessed spectrophotometrically in plasma samples and lipoprotein fractions, using the DiaSys Triglycerides FS kit (ref# 15710, Diagnostic Systems, GmbH) and the Lab Assay phospholipid determination kit (cat# 296-63801, Wako Chemicals, USA), respectively. Total protein in lipoprotein fractions was measured by the Lowry assay using the DCTM Protein Assay Kit (cat# 500-0116, Bio-Rad, USA).
Following four hours of fasting, plasma and serum samples were isolated from the untreated groups of mice. Pooled plasma of 0.4 mL from each mouse group were fractionated by KBr density gradient ultracentrifugation, over a 4 mL KBr (Sigma-Aldrich, St. Louis, USA) gradient (1.23 g/mL over 1.21 g/mL over 1.063 g/mL over 1.019 g/mL over saline), as described previously[22]. For all analyses, equal volumes of all isolated HDL fractions were pooled together, except for the determination of the anti-inflammatory capacity of HDL, where single fractions of endotoxin free serum lipoproteins were fractionated by a different density gradient (1.063 g/mL over 1.019 g/mL over 1.0063 g/mL) and separation of 4 mL of pooled serum samples was performed under aseptic conditions.
Mitochondria from brown adipose tissue (BAT) and white adipose tissue (WAT) were isolated as described previously[23]. The protein concentration of each mitochondrial sample was determined using the DCTM Protein Assay Kit.
For the semiquantitative measurement of human APOA-Ⅱ, and murine APOA-Ⅰ, APOE, APOC-Ⅰ, APOC-Ⅱ and APOC-Ⅲ in lipoprotein fractions, Western blotting analysis was performed. Goat anti-APOA-Ⅱ (cat# K34001G), anti-APOA-Ⅰ (cat# K45252), anti-APOC-Ⅰ (cat# K74110G), anti-APOC-Ⅱ (cat# K59600R) and anti-APOC-Ⅲ (cat# K74140G) antibodies from Meridian Life Science, USA, were used respectively as primary, and a rabbit anti-goat antibody (cat# sc-2768, Santa-Cruz, USA) as secondary. For the detection of APOE rabbit anti-APOE (cat# K23100R, Meridian Life Science) was used as primary and a goat anti-rabbit antibody (cat#7074, Cell Signaling Technology, Danvers, USA) as secondary. Western blotting for cytochrome C (CYTC), uncoupling protein 1 (UCP1), and cytochrome C oxidase subunit 4 (COX4) was performed using rabbit anti-mouse antibodies (cat# 4272, Cell Signaling; cat# GTX10983, Acris, Herford, Germany; cat# 4844, Cell Signaling, respectively). SDS-PAGE of pure mitochondrial extracts was performed using 6 μg protein/sample for BAT and 15 μg protein/sample for WAT. Semiquantitative determination of the relative protein amounts was performed by Image J free software.
Transmission electron microscopy (TEM) analysis of purified HDL particles was performed at the Laboratory of Electron Microscopy and Microanalysis (L.E.M.M.) of the University of Patras (http://www.electronmicroscopylab.upatras.gr/index.php/en/). Briefly, APOA-Ⅱ-HDL particles were visualized by TEM at 200K magnification, then photographed and analyzed. HDL from AdGFP infected mice was used as control. By visual inspection, the distributions of HDL particle diameter and area for the samples were evidently skewed. Image analysis of TEM pictures was performed by Image J free software and was followed by unpaired t-test statistical analysis for comparison of particle diameter between APOA-Ⅱ-HDL and control-HDL samples. A total number of 100 particles were measured per image.
Lipoprotein-associated phospholipase A2 (Lp-PLA2) activity was measured by a modification of the trichloroacetic acid precipitation procedure in plasma using [3H]-PAF (1-O-hexadecyl-2-[3H-acetyl]-sn-glycero-3-phosphocholine) (10 Ci/mmol, DuPont-New England Nuclear, Boston, MA, USA) as a substrate at a final concentration of 100 μmol/L, as described previously[24]. Briefly, 50 μL diluted plasma (1∶25 v/v with HEPES pH7.4 buffer which consists of 4.2 mmol/L HEPES, 137 mmol/L NaCl, 2.6 mmol/L KCl, and 2 mmol/L EDTA) as the source of the enzyme or blanks (for determination of background) were mixed with assay buffer (HEPES) to a final volume of 90 μL. The reaction was allowed to proceed for 10 minutes at 37 °C by addition of 10 μL of working substrate solution (2 mmol/L [3Η]-PAF in 2.5×103 mg/L BSA, prepared fresh daily). The reaction was terminated by addition of 20 μL ice-cold aqueous bovine serum albumin (BSA) solution (100×103 mg/L) followed by vortex-mixing and incubation for 10 minutes at 4 °C. Then, 80 μL of ice-cold trichloroacetic acid solution (20 % TCA) were added and mixture was incubated for an additional 30 minutes at 4 °C. The samples were centrifuged at 6 000 g for 5 minutes at 4 °C and 100 μL οf supernatant were transferred to 2 mL of scintillation fluid. The amount of [3H] tracer was then quantitated in a liquid scintillation counter (Packard Tri-Card 2100). Lp-PLA2 activity was expressed as nmol PAF degraded per mL of plasma per minute as described previously [24].
For the assessment of the anti-oxidant capacity of APOA-Ⅱ-HDL a modification of the dihydrorhodamine 123 (DHR) method[25] was used, as described previously[18]. The oxidation rate of DHR in the presence of APOA-Ⅱ-HDL was assessed. HDL from AdGFP infected mice was used as control. Oxidation rate was calculated for each well as the slope for the linear regression of fluorescence intensity between 10 and 50 minutes and expressed as fluorescence units per minute. The lower the slope, the lower the substrate oxidation rate.
Cholesterol efflux capacity of APOA-Ⅱ-HDL was measured using RAW 264.7 macrophage cell line as described previously[18]. Total [14C]-Cholesterol input was calculated as the sum of [14C]-Cholesterol in the efflux medium plus [14C]-Cholesterol present in the cell lysate. The rate of HDL-stimulated [14C]-Cholesterol efflux was expressed as the ratio of total [14C]-Cholesterol effluxed onto APOA-Ⅱ-HDL and corrected for non-specific [14C] tracer efflux measured in the absence of HDL. HDL from AdGFP infected mice was used as control.
The effects of APOA-Ⅱ-HDL on inflammation were assessed in RAW 264.7 macrophage cell line. Briefly, cells were seeded in 96-well plates (7×104 cells/well) and cultured in DMEM containing 10% FBS and 1% pen/strep. After a 16-hour incubation at 37 °C, cultures were washed with serum-free DMEM medium and treated for 4 hours with lipopolysaccharide (LPS) (100 ng/mL) that was preincubated for 30 minutes at 37 °C with serum HDL. The amount of HDL used in the assay was defined by the total final concentration of HDL proteins added to the incubation medium. The protein concentration of each HDL sample was determined using the DCTM Protein Assay Kit (cat# 500-0116, Bio-Rad). As control, cells were incubated with DMEM containing serum HDL in the absence of exogenous LPS. Following incubation, medium was collected and TNFα was determined by ELISA.
Five days post-infection with AdGFP or AdGFP-APOA-Ⅱ, mice were fasted for 16 hours, GTT and IST analyses were performed and blood glucose determination was performed at the indicated timepoints of each test. Plasma glucose was determined by DiaSys Glucose FS kit (cat# 125009910021, Diagnostic Systems GmbH).
All data sets were tested using the Kolmogorov-Smirnov and the Shapiro-Wilk tests and were treated with parametric (P>0.1) or non-parametric tests (P<0.1) according to their deviation from normality. Data are reported as mean±SEM. All statistical tests were performed using the GraphPad Prism 6 software.
To test the expression and secretion of APOA2 in vitro, we analyzed culture medium from HTB-13 cells infected with AdGFP or AdGFP-APOA-Ⅱ. Analysis of the culture medium by Western blotting confirmed the efficient APOA-Ⅱ secretion at 48 hours post-infection (Fig. 2A).
To confirm human APOA-Ⅱ expression in vivo, C57BL/6 mice were infected with AdGFP or AdGFP-APOA-Ⅱ and five days post-infection, liver and plasma samples were collected. Then total hepatic RNA was analyzed by Northern blotting and plasma by Western blotting for APOA-Ⅱ mRNA and APOA-Ⅱ protein respectively, confirmed the efficient expression of APOA-Ⅱ in vivo (Fig. 2B and C).
The human APOA-Ⅱ protein contains a free cysteine residue capable of forming inter-molecular S-S bonds resulting in APOA-Ⅱ dimers[26]. When expressed in cell cultures, APOA-Ⅱ could be detected at both its monomeric and dimeric forms (Fig. 2A). However, the dimeric form was no longer evident when APOA-Ⅱ was expressed in mice (Fig. 2C).
Analysis of plasma lipid levels five days post-infection showed that expression of APOA-Ⅱ resulted in a significant increase of plasma total cholesterol, triglyceride and phospholipid levels, compared to the same mice prior to infection (day 0) or the AdGFP infected mice five days post-infection (Fig. 3A–C). This increase was associated with a marked increase in chylomicrons/VLDL, IDL and LDL cholesterol triglycerides and phospholipids and a shift of HDL cholesterol distribution towards smaller and more dense HDL particles (Fig. 3D–F). The relative lipid content of APOA-Ⅱ-HDL expressed as mg of total HDL protein or wt% content of total lipids in HDL is shown in Table 1.
Lipid content | per mg of protein | wt% content of total lipids | |||
AdGFP | AdGFP-APOA-Ⅱ | AdGFP | AdGFP-APOA-Ⅱ | ||
Total cholesterol/mg of protein | 0.253±0.007 | 0.208±0.004 | 34.9±0.94 | 33.7±0.63 | |
Triglycerides | 0.030±0.004 | 0.019±0.005 | 4.3±0.56 | 3.2±0.80 | |
Phospholipids | 0.439±0.014 | 0.390±0.015 | 60.8±1.85 | 63.1±2.47 | |
Total lipids | 0.722±0.025 | 0.618±0.006 | 100 | 100 | |
Total protein (mg/L) | 1 330±80 | 1 810±260 | |||
HDL: high-density lipoprotein. |
Fractionation of plasma samples by density gradient ultracentrifugation followed by Western blotting analysis of lipoprotein fractions for human APOA-Ⅱ and murine APOA-Ⅰ, APOE, APOC-Ⅰ, APOC-Ⅱ and APOC-Ⅲ, showed that APOA-Ⅱ expression resulted in qualitative and quantitative changes in apolipoprotein composition of HDL and other lipoprotein classes five days post-infection (Fig. 4). APOA-Ⅱ was distributed among all HDL fractions and was also present in intermediate-density lipoprotein (IDL) and low-density lipoprotein (LDL) subclasses. No measurable levels of APOA-Ⅱ could be found in the chylomicrons/very low-density lipoprotein (CM/VLDL) fraction. Expression of APOA-Ⅱ resulted in substantial recruitment of APOE in HDL, while APOA-Ⅰ expression remained unchanged. Some lipid-free APOE was also visible. Interestingly, APOA-Ⅱ expression also increased the levels of APOCs (APOC-Ⅰ, APOC-Ⅱ and APOC-Ⅲ) though in different lipoprotein fractions. In contrast to the control group, where trace amounts of APOC-I were present and APOC-Ⅱ and APOC-Ⅲ were not detectable, a significant increase in APOC-Ⅱ content was observed in all lipoproteins, while APOC-Ⅰ was significantly augmented in VLDL and LDL. Measurable levels of APOC-Ⅲ were also present mainly in LDL with trace amounts found in HDL.
To study the effects of human APOA-Ⅱ on HDL particle geometry, we next isolated HDL from the plasma of infected mice and performed a qualitative negative staining TEM analysis. As expected, negative TEM staining confirmed the presence of spherical HDL particles in the HDL fractions of plasma from AdGFP infected mice (Fig. 5A). However, similar analysis revealed a significant presence of discoidal (indicated by white arrows) HDL particles in the plasma of mice expressing APOA-Ⅱ (Fig. 5B). Average APOA-Ⅱ-HDL particle diameter, expressed as median (Min to Max) was calculated to be (12.54±0.32) nm, and displayed no statistically significant difference from the average diameter of control HDL, calculated to be (12.42±0.27) nm (Fig. 5C).
Given the distinct structural differences between APOA-Ⅱ-HDL and control-HDL, we next sought to investigate how these differences may influence HDL functionality such as HDL antioxidant activity and total cholesterol efflux from RAW 264.7 macrophage cells in vitro.
As shown in Fig. 6A, APOA-Ⅱ-HDL demonstrated a significantly higher antioxidant function (i.e. greater inhibition of substrate oxidation) compared to control-HDL, when equal amounts of HDL cholesterol from each group were used in the dihydrorhodamine assay. Control-HDL also inhibited substrate oxidation as expected, though to a much lesser extent.
As shown in Fig. 6B, when equal amounts of HDL cholesterol from each group were used in the cholesterol efflux assay, no differences between the capacities of APOA-Ⅱ-HDL and control-HDL to accept [14C]-cholesterol from [14C]-cholesterol-charged RAW 264.7 cells were observed.
To compare the effects of APOA-Ⅱ-HDL on inflammation, we employed the pertinent model of LPS-induced inflammation in RAW 264.7 macrophages, as described previously[18,27]. In the absence of LPS, addition of 10 μg/mL HDL from each tested sample (i.e. APOA-Ⅱ-HDL and control-HDL) did not result in any significant production of TNFα confirming that samples were properly prepared free of LPS. Stimulation of cells with LPS in the absence of HDL resulted in a significant production of TNFα in culture medium. When LPS stimulation was performed in the presence of APOA-Ⅱ-HDL, a significant inhibition of TNFα production was observed compared to control-HDL, which also showed a smaller yet measurable anti-inflammatory effect (Fig. 6C).
As shown in Fig. 6D, expression of APOA-Ⅱ in mice infected with AdGFP-APOA-Ⅱ resulted in a significantly higher plasma Lp-PLA2 activity.
In an attempt to study the effects of APOA-Ⅱ expression on adipose tissue metabolic activity, in the next set of experiments, mitochondria were isolated from BAT and WAT of mice infected with AdGFP-APOA-Ⅱ or AdGFP. In WAT mitochondrial fractions, we found that expression of APOA-Ⅱ stimulated a significant induction of mitochondrial CYTC levels (corrected for COX4 expression), suggesting elevated oxidative phosphorylation (Fig. 7A–C). Moreover, it appeared that oxidative phosphorylation was coupled with respiration towards ATP production, due to an apparent relative decrease of UCP1 when corrected for mitochondrial COX4 (Fig. 7A–C and G).
Similar analysis of BAT mitochondrial fractions showed that APOA-Ⅱ expression did not have any substantial impact on mitochondrial CYTC and UCP1 levels further indicating no effects on oxidative phosphorylation and non-shivering thermogenesis in this tissue (Fig. 7D–F).
To determine the effects of APOA-Ⅱ expression on plasma glucose homeostasis, GTT and IST tests were performed in mice infected with AdGFP-APOA-Ⅱ or AdGFP five days post-infection.
On day 0 (immediately prior to infection), all mouse groups displayed comparable glucose tolerance when GTT was performed (Fig. 8A and C). Similarly, no significant differences in insulin sensitivity between groups were detected when IST was performed (Fig. 8D and F). Moreover, both groups had normal fasting glucose levels between 60–70 mg/dL (Fig. 8G).
On day five post-infection, glucose tolerance of APOA-Ⅱ-expressing mice appeared significantly improved compared to the control group (Fig. 8B and C). Nevertheless, these mice displayed comparable insulin sensitivity to that of mice infected with AdGFP (Fig. 8E and F), indicating an unaffected physiological response to intraperitoneally administered exogenous insulin. Both the control and APOA-Ⅱ-expressing mouse groups showed a tendency towards higher fasting plasma glucose levels, between 80–90 mg/dL, though the increase did not reach statistical significance (Fig. 8G).
Almost 35 years after human APOA-Ⅱ amino acid sequence was decoded[1], our knowledge of APOA-Ⅱ functions in relation to human health and disease remains limited. The sole and most widely accepted function of APOA-Ⅱ is in biogenesis of HDL. In the present study, we sought to investigate the impact of APOA-Ⅱ on HDL structure and function along with its effects on adipose tissue metabolic activity and plasma glucose homeostasis.
Western blotting analysis of lipoprotein fractions showed that expression of APOA-Ⅱ resulted in recruitment of APOE on HDL, while APOA-Ⅰ expression remained unchanged. Interestingly, APOA-Ⅱ expression also increased the levels of APOCs (APOC-Ⅰ, APOC-Ⅱand APOC-Ⅲ) though in different lipoprotein fractions. These increases may be responsible for the observed increase in plasma triglyceride levels of mice expressing APOA-Ⅱ (Fig. 3B). Indeed, APOE and APOCs are known inhibitors of plasma lipoprotein lipase (LPL)[28– 29], while APOE is also known to increase hepatic VLDL-TG production and secretion[30]. This finding agrees with previous data showing that APOA-Ⅱ indirectly influences LPL functionality[31]. Nevertheless, our data cannot preclude direct effects of APOA-Ⅱ on LPL activity and hepatic VLDL-TG production in vivo. Such effects are currently under investigation.
Our results indicated that accumulation of APOA-Ⅱ on HDL, resulted in substantial numbers of discoidal HDL particles (Fig. 5). This finding agrees with previous work that APOA-Ⅱ is an indirect inhibitor of lecithin-cholesterol acyltransferase (LCAT) activity in plasma[32]. This situation is reminiscent of the LCAT-deficient mouse where lack of LCAT results in discoidal HDL particles even though those particles contained primarily APOE and barely detectable levels of APOA-Ⅰ[27]. In the present work, we did not assess the effects of APOA-Ⅱ-HDL on LCAT activity, however, it would be interesting to perform this measurement in the future.
The mean diameter of APOA-Ⅱ-HDL particles is comparable to that of control HDL particles in mice infected with AdGFP, a finding consistent with the data of Table 1, showing no statistically significant difference in the HDL lipids between groups.
Moreover, APOA-Ⅱ expression results in a striking increase in the phospholipid content of VLDL, IDL/LDL and denser HDL. Since many phospholipids (such as sphingosine-1-phosphate for example) are bioactive with important signaling properties, it would be interesting in a future lipidomic study to determine the precise nature and function of these phospholipids.
At this point, it is not clear what drives the effects of APOA-Ⅱ expression on HDL apolipoprotein composition. A likely possibility may include protein-protein interactions on the particle surface, such as in the case of APOA-Ⅱ and APOE[3]. Indeed, the increased presence of APOE on APOA-Ⅱ-HDL could be due to the previously reported dimerization between these two proteins. This way, APOA-Ⅱ could act as an anchor that attracts APOE on HDL. However, one cannot exclude that increased affinity of certain proteins to specific HDL particle biophysical characteristics, such as lipid packing density or surface curvature is responsible. Alternatively, given that APOA-Ⅱ, APOE, APOA-Ⅰ, APOC-Ⅰ, APOC-Ⅱ and APOC-Ⅲ can interact functionally with ABCA1 to form HDL-like particles, it is also possible that APOA-Ⅱ modulates the affinity of ABCA1 for these apolipoproteins. Of note, under our experimental conditions, endogenous APOA-Ⅱ was below detection limit, so the effects of human APOA-Ⅱ on the expression of the murine APOA-Ⅱ and its affinity for HDL could not be assessed.
In an analogy with our previous observations with APOE-, APOA-Ⅰ-, and APOC-Ⅲ-containing HDL[17– 18], here we found that changes in particle apolipoprotein composition brought about by APOA-Ⅱ correlate with significant alterations in particle functionality. APOA-Ⅱ-HDL alleviated the effect of LPS on TNFα release from RAW 264.7 macrophages suggesting an anti-inflammatory role of APOA-Ⅱ-HDL in circulation (Fig. 6C). Similarly, APOA-Ⅱ-HDL had a much higher antioxidant capacity than control-HDL, suggesting a positive role in reducing oxidative stress (Fig. 6A). In contrast, APOA-Ⅱ did not appear to enhance the capacity of HDL to accept [14C]-cholesterol from RAW 264.7 cells (Fig. 6B). When plasma Lp-PLA2 activity was assessed, it was found increased in mice infected with AdGFP-APOA-Ⅱ, indicating that APOA-Ⅱ is rather an activator of this plasma enzyme which under certain circumstances may be considered a risk factor for CHD[33].
Mitochondrial CYTC is a biomarker of mitochondrial metabolic function. In adipose tissue, this function relates to ATP production when oxidative phosphorylation is coupled to respiration or non-shivering thermogenesis, marked by increased mitochondrial UCP1 expression, when oxidative phosphorylation is uncoupled from respiration. Increased thermogenesis is a result of increased mitochondrial metabolism and mainly UCP1 function that mediates the metabolic conversion of free fatty acids to heat through non-shivering thermogenesis. However, induction of WAT mitochondrial oxidative phosphorylation for ATP production, independently of UCP1 increase, may also contribute to the lean phenotype[34]. Our molecular analysis revealed a selective increase of CYTC in WAT, while UCP1 levels were decreased (Fig. 7A–C). These changes indicate that APOA-Ⅱ stimulates oxidative phosphorylation, coupled with respiration for ATP production in WAT, supporting that APOA-Ⅱ may facilitate substrate oxidation and eventually weight loss in these mice. In contrast, APOA-Ⅱ expression did not have any effects on BAT mitochondrial metabolic activity (Fig. 7D–F).
It is possible that the observed changes in HDL functionality and WAT mitochondrial substrate oxidation may not be a direct effect of APOA-Ⅱ but rather an indirect, as a result of the significant apolipoprotein composition change of HDL, brought about by APOA-Ⅱ expression. The latter is supported by our previous data showing that HDL apoproteome dictates its lipidome and both modulate particle functionality[17–18].
When glucose tolerance was assessed, data indicated that APOA-Ⅱ expression resulted in improved glucose tolerance, which is usually inversely related to WAT accumulation (Fig. 8A–C). However, when IST was performed, no differences were observed between groups (Fig. 8D–F), raising the possibility that APOA-Ⅱ does not impact tissue insulin sensitivity but rather stimulates insulin secretion by pancreatic β-islets. These findings from our study where APOA-Ⅱ is expressed transiently in adult mice differ significantly from data obtained in APOA-Ⅱ transgenic mice, showing that APOA-Ⅱ increases body weight gain and reduces glucose tolerance[35]. It is possible that APOA-Ⅱ also triggers developmental effects on adipose tissue metabolism and insulin secretion and utilization in the transgenic mice that may result in these phenotypic differences. It would be interesting to investigate these possibilities further. However, it is notable that administration of APOA-Ⅱ to the adult mouse positively influences glucose tolerance and the precise mechanism should be explored.
In summary, our data indicate that transient expression of APOA-Ⅱ in C57BL/6 mice results in pleiotropic effects with respect to HDL functionality, adipose tissue metabolism and glucose utilization, many of which are beneficial to health.
We would like to thank Dr. Constantinos Tellis for advising us on the Lp-PLA2 activity assay and Ms. Odysseia Savvoulidou for technical assistance. This work was supported financially by the program "Support of Young Investigators" MIS No. 5005458 that was co-financed by the Operational Program "Human Resources Development, Education and Lifelong Learning" and by the European Union (European Social Fund) and Greek national funds.
[1] |
Brewer HB Jr, Lux SE, Ronan R, et al. Amino acid sequence of human apoLp-Gln-Ⅱ (apoA-Ⅱ), an apolipoprotein isolated from the high-density lipoprotein complex[J]. Proc Natl Acad Sci USA, 1972, 69(5): 1304–1308. doi: 10.1073/pnas.69.5.1304
|
[2] |
Pownall HJ, Gillard BK, Gotto AM Jr. Setting the course for apoAⅡ: a port in sight?[J]. Clin Lipidol, 2013, 8(5): 551–560. doi: 10.2217/clp.13.59
|
[3] |
Borghini I, Barja F, Pometta D, et al. Characterization of subpopulations of lipoprotein particles isolated from human cerebrospinal fluid[J]. Biochim Biophys Acta, 1995, 1255(2): 192–200. doi: 10.1016/0005-2760(94)00232-N
|
[4] |
Warden CH, Hedrick CC, Qiao JH, et al. Atherosclerosis in transgenic mice overexpressing apolipoprotein A-Ⅱ[J]. Science, 1993, 261(5120): 469–472. doi: 10.1126/science.8332912
|
[5] |
van't Hooft FM, Ruotolo G, Boquist S, et al. Human evidence that the apolipoprotein A-Ⅱ gene is implicated in visceral fat accumulation and metabolism of triglyceride-rich lipoproteins[J]. Circulation, 2001, 104(11): 1223–1228. doi: 10.1161/hc3601.095709
|
[6] |
Gordon SM, Chung JH, Playford MP, et al. High density lipoprotein proteome is associated with cardiovascular risk factors and atherosclerosis burden as evaluated by coronary CT angiography[J]. Atherosclerosis, 2018, 278: 278–285. doi: 10.1016/j.atherosclerosis.2018.09.032
|
[7] |
Constantinou C, Karavia EA, Xepapadaki E, et al. Advances in high-density lipoprotein physiology: surprises, overturns, and promises[J]. Am J Physiol Endocrinol Metab, 2016, 310(1): E1–E14. doi: 10.1152/ajpendo.00429.2015
|
[8] |
Tsompanidi EM, Brinkmeier MS, Fotiadou EH, et al. HDL biogenesis and functions: role of HDL quality and quantity in atherosclerosis[J]. Atherosclerosis, 2010, 208(1): 3–9. doi: 10.1016/j.atherosclerosis.2009.05.034
|
[9] |
Barter PJ, Caulfield M, Eriksson M, et al. Effects of torcetrapib in patients at high risk for coronary events[J]. N Engl J Med, 2007, 357(21): 2109–2122. doi: 10.1056/NEJMoa0706628
|
[10] |
Schwartz GG, Olsson AG, Abt M, et al. Effects of dalcetrapib in patients with a recent acute coronary syndrome[J]. N Engl J Med, 2012, 367(22): 2089–2099. doi: 10.1056/NEJMoa1206797
|
[11] |
Lincoff AM, Nicholls SJ, Riesmeyer JS, et al. Evacetrapib and cardiovascular outcomes in high-risk vascular disease[J]. N Engl J Med, 2017, 376(20): 1933–1942. doi: 10.1056/NEJMoa1609581
|
[12] |
The AIM-HIGH Investigators. Niacin in patients with low HDL cholesterol levels receiving intensive statin therapy[J]. N Engl J Med, 2011, 365(24): 2255–2267. doi: 10.1056/NEJMoa1107579
|
[13] |
The HPS2-THRIVE Collaborative Group. Effects of extended-release niacin with laropiprant in high-risk patients[J]. N Engl J Med, 2014, 371(3): 203–212. doi: 10.1056/NEJMoa1300955
|
[14] |
Bowe B, Xie Y, Xian H, et al. High density lipoprotein cholesterol and the risk of all-cause mortality among U.S. Veterans[J]. Clin J Am Soc Nephrol, 2016, 11(10): 1784–1793. doi: 10.2215/CJN.00730116
|
[15] |
Loscalzo J. Molecular mechanisms of atherosclerosis[M]. London: CRC Press, 2004: 111–174.
|
[16] |
Kavo AE, Rallidis LS, Sakellaropoulos GC, et al. Qualitative characteristics of HDL in young patients of an acute myocardial infarction[J]. Atherosclerosis, 2012, 220(1): 257–264. doi: 10.1016/j.atherosclerosis.2011.10.017
|
[17] |
Filou S, Lhomme M, Karavia EA, et al. Distinct roles of apolipoproteins A1 and E in the modulation of high-density lipoprotein composition and function[J]. Biochemistry, 2016, 55(27): 3752–3762. doi: 10.1021/acs.biochem.6b00389
|
[18] |
Zvintzou E, Lhomme M, Chasapi S, et al. Pleiotropic effects of apolipoprotein C3 on HDL functionality and adipose tissue metabolic activity[J]. J Lipid Res, 2017, 58(9): 1869–1883. doi: 10.1194/jlr.M077925
|
[19] |
Pamir N, Pan C, Plubell DL, et al. Genetic control of the mouse HDL proteome defines HDL traits, function, and heterogeneity[J]. J Lipid Res, 2019, 60(3): 594–608. doi: 10.1194/jlr.M090555
|
[20] |
Kontush A, Lindahl M, Lhomme M, et al. Structure of HDL: particle subclasses and molecular components[M]//von Eckardstein A, Kardassis D. High density lipoproteins. Cham: Springer, 2015: 3–51.
|
[21] |
Boyce G, Button E, Soo S, et al. The pleiotropic vasoprotective functions of high density lipoproteins (HDL)[J]. J Biomed Res, 2018, 32(3): 164–182.
|
[22] |
Kypreos KE. ABCA1 promotes the de novo biogenesis of apolipoprotein CⅢ-containing HDL particles in vivo and modulates the severity of apolipoprotein CⅢ-induced hypertriglyceridemia[J]. Biochemistry, 2008, 47(39): 10491–10502. doi: 10.1021/bi801249c
|
[23] |
Constantinou C, Mpatsoulis D, Natsos A, et al. The low density lipoprotein receptor modulates the effects of hypogonadism on diet-induced obesity and related metabolic perturbations[J]. J Lipid Res, 2014, 55(7): 1434–1447. doi: 10.1194/jlr.M050047
|
[24] |
Tselepis AD, Dentan C, Karabina SAP, et al. PAF-degrading acetylhydrolase is preferentially associated with dense LDL and VHDL-1 in human plasma. Catalytic characteristics and relation to the monocyte-derived enzyme[J]. Arterioscler Thromb Vasc Biol, 1995, 15(10): 1764–1773. doi: 10.1161/01.ATV.15.10.1764
|
[25] |
Kelesidis T, Currier JS, Huynh D, et al. A biochemical fluorometric method for assessing the oxidative properties of HDL[J]. J Lipid Res, 2011, 52(12): 2341–2351. doi: 10.1194/jlr.D018937
|
[26] |
Knott TJ, Priestley LM, Urdea M, et al. Isolation and characterisation of a cDNA encoding the precursor for human apolipoprotein AⅡ[J]. Biochem Biophys Res Commun, 1984, 120(3): 734–740. doi: 10.1016/S0006-291X(84)80168-0
|
[27] |
Petropoulou PI, Berbée JFP, Theodoropoulos V, et al. Lack of LCAT reduces the LPS-neutralizing capacity of HDL and enhances LPS-induced inflammation in mice[J]. Biochim Biophys Acta, 2015, 1852(10): 2106–2115. doi: 10.1016/j.bbadis.2015.07.010
|
[28] |
Rensen PCN, van Berkel TJC. Apolipoprotein E effectively inhibits lipoprotein lipase-mediated lipolysis of chylomicron-like triglyceride-rich lipid emulsions in vitro and in vivo[J]. J Biol Chem, 1996, 271(25): 14791–14799. doi: 10.1074/jbc.271.25.14791
|
[29] |
Larsson M, Allan CM, Jung RS, et al. Apolipoprotein C-III inhibits triglyceride hydrolysis by GPIHBP1-bound LPL[J]. J Lipid Res, 2017, 58(9): 1893–1902. doi: 10.1194/jlr.M078220
|
[30] |
Kypreos KE, Van Dijk KW, Havekes LM, et al. Generation of a recombinant apolipoprotein E variant with improved biological functions: hydrophobic residues (LEU-261, TRP-264, PHE-265, LEU-268, VAL-269) of apoE can account for the apoE-induced hypertriglyceridemia[J]. J Biol Chem, 2005, 280(8): 6276–6284. doi: 10.1074/jbc.M413458200
|
[31] |
Julve J, Escolà-Gil JC, Rotllan N, et al. Human apolipoprotein A-Ⅱ determines plasma triglycerides by regulating lipoprotein lipase activity and high-density lipoprotein proteome[J]. Arterioscler Thromb Vasc Biol, 2010, 30(2): 232–238. doi: 10.1161/ATVBAHA.109.198226
|
[32] |
Marzal-Casacuberta A, Blanco-Vaca F, Ishida BY, et al. Functional lecithin: cholesterol acyltransferase deficiency and high density lipoprotein deficiency in transgenic mice overexpressing human apolipoprotein A-Ⅱ[J]. J Biol Chem, 1996, 271(12): 6720–6728. doi: 10.1074/jbc.271.12.6720
|
[33] |
Rosenson RS, Stafforini DM. Modulation of oxidative stress, inflammation, and atherosclerosis by lipoprotein-associated phospholipase A2[J]. J Lipid Res, 2012, 53(9): 1767–1782. doi: 10.1194/jlr.R024190
|
[34] |
Flachs P, Rossmeisl M, Kuda O, et al. Stimulation of mitochondrial oxidative capacity in white fat independent of UCP1: a key to lean phenotype[J]. Biochim Biophys Acta, 2013, 1831(5): 986–1003. doi: 10.1016/j.bbalip.2013.02.003
|
[35] |
Castellani LW, Goto AM, Lusis AJ. Studies with apolipoprotein A-Ⅱ transgenic mice indicate a role for HDLs in adiposity and insulin resistance[J]. Diabetes, 2001, 50(3): 643–651. doi: 10.2337/diabetes.50.3.643
|
1. | Lv Y, Xu L, He Z, et al. The association between pregnancy levels of blood lipids and the risk of preterm birth. Sci Rep, 2024, 14(1): 10800. DOI:10.1038/s41598-024-61119-x |
2. | Fuior EV, Zvintzou E, Filippatos T, et al. Peroxisome Proliferator-Activated Receptor α in Lipoprotein Metabolism and Atherosclerotic Cardiovascular Disease. Biomedicines, 2023, 11(10): 2696. DOI:10.3390/biomedicines11102696 |
3. | Zvintzou E, Xepapadaki E, Skroubis G, et al. High-Density Lipoprotein in Metabolic Disorders and Beyond: An Exciting New World Full of Challenges and Opportunities. Pharmaceuticals (Basel), 2023, 16(6): 855. DOI:10.3390/ph16060855 |
4. | Florea G, Tudorache IF, Fuior EV, et al. Apolipoprotein A-II, a Player in Multiple Processes and Diseases. Biomedicines, 2022, 10(7): 1578. DOI:10.3390/biomedicines10071578 |
5. | Thakkar H, Vincent V, Roy A, et al. Determinants of high-density lipoprotein (HDL) functions beyond proteome in Asian Indians: exploring the fatty acid profile of HDL phospholipids. Mol Cell Biochem, 2022, 477(2): 559-570. DOI:10.1007/s11010-021-04304-0 |
6. | Huang J, Yang Y, He P. Serum apolipoprotein A-II and alpha-2-antiplasmin levels in midtrimester can be used as predictors of preterm delivery. J Int Med Res, 2020, 48(9): 300060520952280. DOI:10.1177/0300060520952280 |
Lipid content | per mg of protein | wt% content of total lipids | |||
AdGFP | AdGFP-APOA-Ⅱ | AdGFP | AdGFP-APOA-Ⅱ | ||
Total cholesterol/mg of protein | 0.253±0.007 | 0.208±0.004 | 34.9±0.94 | 33.7±0.63 | |
Triglycerides | 0.030±0.004 | 0.019±0.005 | 4.3±0.56 | 3.2±0.80 | |
Phospholipids | 0.439±0.014 | 0.390±0.015 | 60.8±1.85 | 63.1±2.47 | |
Total lipids | 0.722±0.025 | 0.618±0.006 | 100 | 100 | |
Total protein (mg/L) | 1 330±80 | 1 810±260 | |||
HDL: high-density lipoprotein. |